Promoting reactive oxygen species generation: a key strategy in nanosensitizer-mediated radiotherapy
Abstract
The radiotherapy enhancement effect of numerous nanosensitizers is based on the excessive production of reactive oxygen species (ROS), and only a few systematic reviews have focused on the key strategy in nanosensitizer-mediated radiotherapy. To clarify the mechanism underlying this effect, it is necessary to understand the role of ROS in radiosensitization before clinical application. Thus, the source of ROS and their principle of tumor inhibition are first introduced. Then, nanomaterial-mediated ROS generation in radiotherapy is reviewed. The double-edged sword effect of ROS and the potential dangers they may pose to cancer patients are subsequently addressed. Finally, future perspectives regarding ROS-regulated nanosensitizer applications and development are discussed.
Radiotherapy (RT) was first reported to be applied in cancer treatment in 1896 [1]. After more than 100 years of development, RT has become one of the main clinical treatments for many types of cancer. Although many new approaches have emerged, approximately 50% of patients suffering from cancer still need RT [2]. RT includes ionizing radiation (IR), such as x-rays and γ-rays, and particle radiation, such as α-rays and β-rays [3,4]. RT can be used alone or as an adjuvant to other treatments. The combination of preoperative or postoperative RT and surgery is commonly used in clinical settings and greatly improves patient overall survival [5]. RT is also used to relieve the suffering of incurable patients with advanced cancer who are not suitable candidates for surgery [6].
However, the inherent limitations of RT make this type of therapy a double-edged sword. High-dose IR damages the healthy tissues around the tumor and causes serious side effects, including gastrointestinal damage and secondary malignancy [7]. Recent studies have shown that radiosensitizers can increase the IR sensitivity of tumor cells to achieve a similar therapeutic efficiency with a relatively low dose of radiation, which represents a promising method for reducing RT-related side effects.
Nanomaterials have generated great interest because of their excellent physical and chemical properties, such as the simplicity of synthesizing nanomaterials with ideal sizes and components, their favorable biocompatibility and biodegradability and their specific targeting ability due to the enhanced permeation and retention effect [8–11]. In RT application, the principle of radiosensitization mediated by nanomaterials has been explicitly classified into the following activities: accelerating IR energy deposition, catalyzing reactive oxygen species (ROS) generation, manipulating the tumor microenvironment and regulating the cell cycle/signaling pathways, among which ROS generation might be the most extensively studied [12]. ROS are broadly described as chemical species derived from molecular oxygen and can be divided into two types: free radical and nonradical species. O2•- and •OH are common free radicals in biological systems, whereas H2O2 and 1O2 are typical nonradical species [13]. Specific molecules included in ROS have been clearly overviewed by Sies et al. [14]. Compared with normal tissues, tumors exhibit an elevated intracellular ROS level due to their high metabolism and mitochondrial dysfunction [15]. Once ROS levels exceed a certain threshold, excessive ROS can induce a tumor-killing effect via oxidative damage to biomolecules and the activation of various cell death pathways, such as apoptosis and autophagy [16]. Numerous therapeutic strategies based on ROS cytotoxicity have been proven effective for killing cancer cells and inhibiting tumor growth. It is therefore a promising strategy to develop ROS-promoting nanomaterials that can enhance the curative effect of RT. However, systematic summaries of nanoradiosensitizing materials and radiosensitizing methods based on the generation of ROS are still rare.
In this review, the authors first introduce the antitumor mechanism of ROS. Second, the authors summarize three existing ways in which nanosensitizers can promote ROS generation. Then, the authors discuss the hidden dangers inherent in the strategy of generating ROS. Finally, the authors further discuss the problems to be solved in this field and the prospects of RT. The review is expected to explain the role of ROS generation in nanosensitizer-mediated tumor RT and contribute to the development of more effective nanosensitizers.
Mechanism of ROS-induced cancer cell death
Physiologically, endogenous ROS are mainly derived from the electron transport chain in the mitochondria or oxidases distributed at diverse sites in cells, such as NADPH oxidases, cytochrome P450 oxidases and monoamine oxidases. In RT, the radiation (x-ray is a commonly used form) interacts with water molecules, which are the main components of cells, to form H2O+ and a free electron. These products then react with another water molecule to form highly active •OH or other ROS species [13]. Tumor inhibition via the generation of ROS is one of the principles of RT. Although the level of ROS in cancer cells is higher and the antioxidant mechanism is strengthened, if ROS are increased to a sufficiently toxic level, the cell structure will be destroyed and different cell death pathways will be activated (Figure 1), achieving the goal of inhibiting tumor growth.
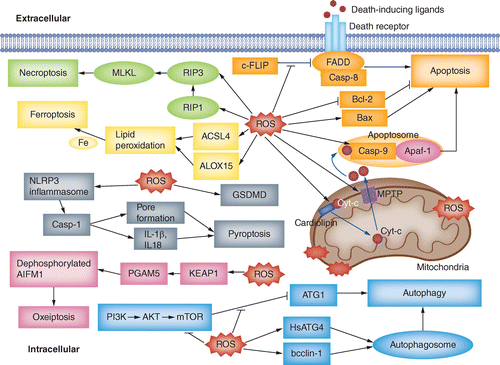
ROS negatively regulate c-FLIP and thereby promote the extrinsic pathway of apoptosis. ROS promote Cyt-c release and MPTP opening, assist in apoptosome formation and regulate the expression of Bcl-2 family proteins, promoting the intrinsic pathway of apoptosis. Also, ROS suppress mTOR activity and enhance the formation of autophagosomes, promoting autophagy. Moreover, ROS increase the levels of RIP3 and RIP1, promoting necroptosis. Accompanied by increases in ACSL4 and ALOX15, ROS lead to lipid peroxidation, which in turn induces ferroptosis. Furthermore, the production of ROS activates the NLRP3 inflammasome and accelerates the cleavage of GSDMD, promoting pyroptosis. ROS can also induce oxeiptosis driven by the KEAP1–PGAM5–AIFM1 pathway.
ROS: Reactive oxygen species.
ROS directly damage biomolecules
ROS, especially •OH (with high activity), attack double-bonded unsaturated fatty acids and generate peroxidized lipids (LOOH), which can trigger and increase cell damage [17]. ROS and the lipid oxidation products can directly interact with DNA bases, resulting in purine and pyrimidine changes and single-strand or double-strand breaks. ROS can cause peptide chain breakage, protein cross-linking and damage to the side chains of branched amino acids or even the advanced structure of proteins through amino acid oxidation, resulting in enzyme inactivation [18]. ROS attack the membranes and proteins of mitochondria, resulting in the disruption of the electron transport chain, opening of the MPTP, collapse of membrane potential and disturbance of ATP synthesis. Longer MPTP opening may lead to ROS outbreaks, which spread between mitochondria, and the cells themselves will ultimately be destroyed [19].
ROS act as second messengers triggering multiple cell death
In the extrinsic pathway of apoptosis, after binding to extracellular ligands, death receptors recruit an adaptor protein and pro-caspase-8 to the cell membrane to form a DISC, subsequently initiating a downstream cascade reaction. ROS downregulate c-FLIP, a redox-sensitive protein that inhibits the formation of DISC via a ubiquitin–proteasomal function, subsequently promoting Fas-mediated apoptosis [20]. The intrinsic pathway starts with an increase in mitochondrial membrane permeability and the release of Cyt-c through the MPTP, after which the released Cyt-c combines with Apaf-1 and pro-caspase-9 to form apoptosomes. In the mitochondria, the oxidation of cardiolipin via ROS is necessary for the release of Cyt-c [21]. When ROS production is suppressed, MPTP opening is significantly blocked, preventing the subsequent release of Cyt-c and caspase-3 activation [22]. ROS also promote apoptosome formation via the oxidative modification of caspase-9, facilitating its interaction with Apaf-1 and promoting its self-cleavage and activation [23]. Moreover, ROS increase the level of proapoptotic proteins (e.g., Bad and Bax) and reduce the expression of antiapoptotic proteins (e.g., Bcl-2) by regulating the phosphorylation and ubiquitination of Bcl-2 family proteins [24].
Autophagy is a process regulated by a series of autophagy-related genes, and the induction of autophagy may be a promising therapeutic strategy for cancer [25]. In normal cells, mTOR acts as a critical inhibitor of autophagy by inhibiting the kinase activity of ATG-1 [26]. ROS can cause conformational changes to ATM via the oxidation of sulfhydryl groups and suppress downstream mTOR activity [27]. Moreover, in cancer cells, the PI3K/AKT/mTOR signaling pathway is often excessively activated, which leads to decreased autophagy and uncontrolled cell growth. ROS can induce autophagy and cell cycle arrest by suppressing the PI3K/AKT/mTOR pathway and ultimately inhibit cancer growth in various types of cancer cells [28,29]. ROS can also promote the formation of autophagosomes by activating the cysteine protease HsAtg4 and increasing the expression of the tumor suppressor beclin-1 [30,31].
Necroptosis is a kind of regulated necrosis dependent on RIP1 and executed by activated RIP3 and its phosphorylated substrate, and the role of necroptosis in ROS-mediated cancer treatments has been illustrated by Hsu et al. [32]. ROS activate RIP1 phosphorylation, which is essential for RIP1 to recruit RIP3 and form necrosomes [33]. ROS can increase the level of RIP3 protein and accelerate the formation of RIP1/RIP3 necrosomes in glioma cells [34].
Ferroptosis is a newly recognized form of regulated cell death dependent on the existence of iron and ROS [35]. ROS derived from IR can remove e- from polyunsaturated fatty acids (PUFA) to generate fatty acid radicals, after which lipid hydroperoxides (PUFA-OOH) is finally formed via a process involving molecular oxygen, which is crucial in driving ferroptosis, and this process is accompanied by the upregulation of ACSL4 and ALOX15 [36].
Pyroptosis is a form of inflammatory regulated cell death, and its process has been reviewed by Miao et al. [37]. ROS activate the NLRP3 inflammasome, leading to the activation of downstream reactions [38]. GSDMD is an essential targeting protein through which ROS regulate pyroptosis via the oxidative modification of four crucial cysteines, and an increasing level of ROS increases the cleavage of GSDMD by caspase-1 [39].
In oxeiptosis, a newly discovered ROS-induced cell death pathway, the sensor KEAP1 induces a specific response according to the concentration of ROS. PGAM5 is released by KEAP1 in the presence of high levels of ROS, leading to the dephosphorylation of AIFM1 and downstream oxeiptosis [40]. However, its role in tumor inhibition is still unknown.
Nanomaterials enhance the RT effect via ROS generation
As mentioned earlier, a high level of toxic ROS can destroy cell structure and activate several cell death pathways to achieve antitumor effects. A wide range of treatments, such as chemotherapeutic drugs and photodynamic therapy, are based on the production of high levels of ROS. Therefore, promoting the generation of toxic ROS in RT is a promising sensitization strategy, and a series of nanomaterials have been proven to have the capacity for RT enhancement. Here the authors summarize the principles of catalyzing ROS production as follows (Figure 2). First, high-Z nanosensitizers enhance the photoelectric effect and increase the emission of secondary electrons. Second, transition metal-based nanosensitizers catalyze the Haber–Weiss and Fenton reactions. Third, semiconducting nanosensitizers form highly reactive electron–hole pairs under x-ray excitation. In this section, the authors introduce the ROS catalytic mechanism of nanosensitizers and provide examples to illustrate their application in RT sensitization. Typical studies are listed in Table 1.
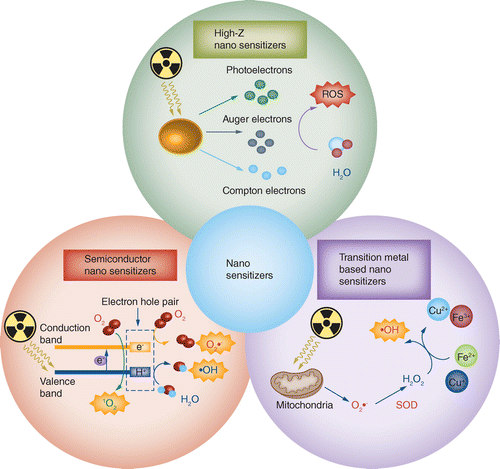
High-Z nanosensitizers enhance the photoelectric effect and increase the emission of secondary electrons. Transition metal-based nanosensitizers catalyze the Haber–Weiss and Fenton reactions. Semiconductor nanosensitizers form highly reactive electron–hole pairs.
ROS: Reactive oxygen species.
Nanosensitizers | Cell line/model | Radiation source and dose | ROS generation principle | Therapeutic effect | Ref. |
---|---|---|---|---|---|
Au NPs | Human cervical cancer (HeLa) cell line | γ-ray, 10 Gy | e- transfer from the surface to O2 to form O2•- | Sensitization enhancement ratio of 5.18 and higher induction of apoptosis were detected | [41] |
Au8NCs | EC1 cells BALB/c nude mice | X-ray, 2 and 4 Gy | Catalysis of ROS production by the electroactive surface | Cell survival decreased to 2.7% Tumor growth was inhibited by 74.2% | [42] |
Ag NPs | U251 cells | X-ray, 4 Gy | Enhanced H2O radiolysis | Cell viability decreased with an increasing concentration of Ag NPs (below 20% at 0.8 mM) | [43] |
Gd oxide nanocrystals | A549, NH1299 and NH1650 cells | Carbon ion radiation, 0.5 and 2 Gy | Facilitation of secondary electron production and increased H2O radiolysis | Maximum SER (10% cell survival fraction) values were 1.10 (A549), 1.11 (NH1299) and 1.20 (NH1650) | [44] |
CuO NPs | MCF-7 cells Mice bearing murine cervical U14 tumors | X-ray, 2, 4, 6 and 8 Gy | Copper ion reaction with H2O2 (Fenton-type reaction) | Cell survival decreased with an increasing radiation dose and cell index dropped below 0.8 (a.u.) Relative tumor volume and tumor weight decreased to approximately 5 (V/V0) and 0.6 (g), respectively | [45] |
Iron oxide NPs | A549 cells | X-ray, 5 Gy | Iron ion participation in the catalysis of the Haber–Weiss reaction | Relative viability of the Fe3O4+radiation group at day 7 was approximately 0.5 | [46] |
ZnO nanorods | SMMC-7721 cells BALB/c nude mice | X-ray, 3 Gy | Photocatalytic properties | Killing effect of ZnO was dose-dependent and cell viability was below 60% (100 μg/ml) Relative tumor volume of TfR/Dox/ZnO nanocomposite group declined to approximately 30% | [47] |
PAA-TiOx NPs | MIAPaCa-2 human pancreatic cancer cell line BALB/c nude mice | X-ray, 0–6 Gy | Phototoxic effects of TiO2 NPs | Cells treated with PAA-TiOxNPs and x-rays showed higher γ-H2AX focus formation and lower surviving fraction Tumor volume of mice treated with PAA-TiOxNPs and x-rays reduced to 35.4% compared with mice undergoing treatment with x-rays alone | [48] |
Gd-doped ZnO NPs | SKLC-6 cells | X-ray, 2, 4, 6 and 8 Gy | Increased absorption of radiation and photocatalytic properties | SER values were 1.47 (10 μg/ml) and 1.61 (20 μg/ml) | [49] |
Au-Bi2S3 NPs | HeLa cells, 4T1 cells and HUVECs BALB/c female nude mice | X-ray, 50 kV, 75 μA X-ray, 6 Gy | The Schottky barrier between Au and Bi2S3 traps e- and transfers them to Au, leading to efficient separation of the electron–hole pairs | Treatment with Au-Bi2S3s and x-rays resulted in an apparent decline in cell viability to 8.68% in HeLa cells, and cell viability of HUVECs under the same treatment was 71.59% Antitumor rate of Au-Bi2S3-induced RT reached 76.47% compared with control | [50] |
GQDs | SW620 and HCT116 cell lines | γ-ray, 3 and 6 Gy | Energy and e- transfer produce 1O2; electron–hole pairs produce •OH and O2•- | Cell growth rates decreased (GQDs + 3 Gy) and cell colony numbers in GQDs + 3 Gy group were lower than those in the 3 Gy group | [51] |
High-Z element-based nanosensitizers facilitate ROS generation by enhancing the photoelectric effect
Photoelectric absorption and secondary electrons derived from IR can produce ROS in RT [52]. High-atomic-number (high-Z) element-based nanosensitizers can absorb more photons under radiation because of their very large capture cross-section, and more energy is deposited in high-Z nanomaterials than in soft tissue, which is mainly composed of low-Z elements [53]. Nanosensitizers also act as a second enhanced source of emissions, including photoelectrons, Compton electrons and Auger electrons, which react with oxygen-containing substances, such as water or oxygen in the surrounding medium and produce ROS [54]. Many studies have focused on improving the high-Z nanomaterial ROS production capacity to reduce the dependence on high-dose radiation.
Nanomaterials based on gold (Au) (Z = 79) are markedly attractive in studies on radiosensitizers, which is attributed to beneficial characteristics, including a simplified synthesis process, controllable size and shape, good biocompatibility and ease of surface modification [55,56]. The detailed mechanism of the tumor-killing effect of Au nanoparticles (NPs) under γ-radiation was first discovered by Yadav et al., who found that elevated ROS levels induced by Au NPs efficiently kill tumors by blocking cell proliferation and inducing apoptosis [41]. In further research, the efficiency of ROS generation induced by Au was found to be related to the size and shape of Au materials. For example, Au8 nanoclusters with ultrasmall sizes exhibit a stronger ability to adsorb, emit and scatter radiation, leading to remarkable sensitizing effects both in vitro (Figure 3A & B) and in vivo (Figure 3C), which can be attributed to a larger ROS burst under x-ray radiation (Figure 3D), followed by irreversible apoptosis [42]. In addition to the size effect, the shape effect of Au nanosensitizers has a great influence on ROS generation. Compared with nanospikes and nanorods, NP-based Au sensitizers generate more ROS, and the order of ROS levels (NPs >nanospikes >nanorods) is consistent with their sensitization enhancement ratio order (1.62, 1.37 and 1.21) [57]. However, Au nanosensitizers exhibit some disadvantages, such as degree of cytotoxicity, low cellular uptake and, consequently, low ROS generation. Hence, several surface modifications have been applied to compensate for the aforementioned deficiencies; for example, thio-glucose-bound Au NPs, radiation-responsive PEGylated Au NPs and Au NPs modified with cell-penetrating peptides (such as TAT peptides) have been found to effectively increase cellular uptake and ROS-mediated tumor cell proliferation inhibition [58–60].

(A) Schematic representation of the synthesis of Au8NCs (orange represents Au, red represents O, gray represents C, turquoise represents Cl and yellow represents S) and Au8NCs used for cancer RT via the induction of ROS production. (B) The live/dead imaging and statistical results of colony formation assays as well as wound healing assay of human esophageal squamous cancer cells (EC1) after different treatments. (C) Dissected tumors after different treatments. (D) Intracellular ROS imaging of EC1 cells at 6 h after different treatments.
Au: Gold; NC: Nanocluster; ROS: Reactive oxygen species; RT: Radiotherapy.
Reproduced with permission from [42], © American Chemical Society (2019).
Silver (Ag) (Z = 47) NPs (Ag NPs) are another type of nanomaterial that have been explored for use in radiosensitization because of their high-Z character, appropriate surface and broad-spectrum antimicrobial function [61]. Although it has been proven that Ag NPs enhance RT effectiveness in malignant glioma both in vitro and in vivo, the cell inhibition mechanism remains unknown. Wu et al. discovered that the radiosensitization effect of Ag NPs was mainly attributed to ROS effects and subsequent apoptosis [43]. Ag NPs can be coupled with cetuximab, a humanized monoclonal antibody targeting EGFR, to achieve combined radiosensitization and targeted therapy for nasopharyngeal carcinoma [62]. However, the toxicity of Ag NPs should be carefully monitored because Ag NPs easily release Ag ions in oxidative surroundings, which affects RNA transcription [63].
In addition to Au and Ag, several other high-Z element-based nanomaterials, such as cerium (Z = 58) [64], gadolinium (Gd) (Z = 64) [44], hafnium (Z = 72) [65,66], tungsten (Z = 74) [67], iridium (Z = 77) [68], platinum (Pt) (Z = 78) [69] and bismuth (Bi) (Z = 83) [70], show the potential to generate ROS to improve the outcome of RT. Here the authors describe some typical paradigms to illustrate nanomaterial-mediated radiosensitization. Based on the fact that Pt accelerates water ionization and radical generation, Salehi et al. prepared a platinum mesoporous nanostructure that improved killing efficacy upon x-ray radiation and decreased melanoma cell viability to approximately 1% when combined with radiation (laser + x-ray) [69]. Li et al. synthesized Gd-based Gd oxide nanocrystals, which induced an increase in •OH production and decreased non-small-cell lung cancer cell survival under carbon ion radiation [44]. Neufeld et al. synthesized a hafnium-based nanoscale metal–organic framework containing the ligand 1,4-dicarboxybenzene, which efficiently increased the production of •OH, O2•- and 1O2 under x-ray radiation and exerted a strong radiation enhancement effect on 4T1 breast cancer cells [65]. Because of the high probability of radiation interaction and ROS accumulation, bismuth NPs have been seen as promising novel radiosensitizers. However, more interaction also means that bismuth NPs are more prone to suffer structural damage under radiation. Zhou et al. introduced graphene nanosheets to inhibit the radiation corrosion of bismuth NPs and reduce the consumption of ROS [71]. Moreover, radiation-excited e- react with high-level H2O2 in cancer cells to produce more ROS, greatly improving the effect of RT.
Transition metal-based nanosensitizers facilitate ROS generation via the catalysis of the Haber–Weiss & Fenton reactions
RT increases the leakage of e- from the electron transport chain and increases the mitochondrial production of O2•-, which is converted to H2O2 by superoxide dismutase. Then, some transition metal-based sensitizers can catalyze H2O2 conversion via the Fenton reaction or the Haber–Weiss reaction. Through these processes, excessive H2O2 produced by cellular metabolism will be catalyzed by transition metal ions (e.g., Fe2+/Fe3+) to form more toxic, highly active •OH or O2•-, which markedly increases the tumor-killing effect of RT [72]. Klein et al. explored the RT enhancement of superparamagnetic iron oxide NPs, which are monocrystalline NPs containing a magnetite (Fe3O4) or maghemite (γFe2O3) structure, and found that surface iron ions can act as catalysts of the Haber–Weiss cycle [73]. Moreover, to produce more available ions under x-ray radiation, they designed incompletely citrate-coated superparamagnetic iron oxide NPs that increased ROS generation by approximately 240% compared with that seen in cells without superparamagnetic iron oxide NPs. Furthermore, the researchers modified the surface of Fe3O4 and CoFe2O4 NPs with self-assembled, monolayer-forming compounds to increase the stability and biocompatibility of Fe3O4 and CoFe2O4 NPs. Activated surfaces containing Fe2+ and Co2+ ions efficiently promoted ROS production via the Fenton reaction under low-dose radiation in MCF-7 cells [74]. To avoid lysosomal encapsulation and promote •OH formation, Hauser et al. conjugated TAT, a cell-penetrating peptide, to iron oxide NPs. These nanoplatforms increased radiation-induced ROS generation and damaged mitochondrial integrity in A549 cells [46]. Based on the fact that FePt NPs can release Fe2+ and produce ROS, Peng et al. designed multifunctional GO/5-fluorouracil–metronidazole nanocomposites. With GO as the skeleton, FePt NPs, the cytotoxic drug 5-fluorouracil and metronidazole were loaded to achieve combined RT and chemotherapy [75].
Copper ions can also react with endogenous H2O2 to generate O2•- or •OH via a Fenton-type reaction [76]. Jiang et al. synthesized CuO NPs (Figure 4A) and observed increased inhibition of MCF-7 cells in the presence of CuO NPs and x-ray radiation (Figure 4C), accompanied by an elevated ROS level (Figure 4B) [45]. The increased antitumor efficacy may result from excessive autophagy. The in vivo experiments also demonstrated radio enhancement and encouraging biosafety of CuO NPs (Figure 4D). Du et al. fabricated TPGS-functionalized Cu3BiS3 nanocrystals for image-guided enhanced RT [77]. Copper ions on the surface of TPGS-functionalized Cu3BiS3 nanocrystals can produce highly reactive •OH and enhance the cancer-killing effect under radiation both in vitro and in vivo.

(A) Schematic illustration of the synthetic route and RT sensitization effect of CuO NPs. (B) Flow cytometric results for ROS generation in MCF-7 cells. (C) Real-time growth results for MCF-7 cells after each treatment. (D)In vivo experiments: relative tumor volumes (a) and body weights (b) after different treatments; (c) tumor weights after different treatments of tumors at day 14; and (d) biodistribution of Cu in mice treated with CuO NPs at days 1 and 14.
NP: Nanoparticle; ROS: Reactive oxygen species; RT: Radiotherapy.
Reproduced with permission from [45], © American Chemical Society (2019).
Semiconducting sensitizers facilitate ROS generation via the formation of electron–hole pairs
First, semiconductor nanomaterials were found to have the capacity to generate ROS under UV radiation [78]. If the energy level of the light is higher than the band gap, photons will be absorbed, and then e- in the valence band will be excited into the conduction band, forming a hole (h+) in the valence band [79]. The highly reactive electron–hole pairs are then transferred to the surface of the materials [80], which facilitates the generation of ROS: O2 accepts e- (forming O2•-), whereas h+ may oxidize H2O (forming HO•). Moreover, the radiative recombination of electron–hole pairs may lead to the emission of photons that excite ground state O2 to excited state 1O2 [81]. However, UV disappointingly fails to penetrate human tissues and reach the internal organs because of the excitation wavelength, which limits its clinical application [82]. As the frequency of x-rays seems to make their application an ideal alternative excitation method for the formation of electron–hole pairs [83], researchers have attempted to design semiconducting sensitizers to generate ROS under x-ray radiation to enhance the effect of RT.
Metal-based semiconductors
Some metal oxides/sulfides, such as ZnO, TiO2, ZnS [84] and WS2 [85], have shown the potential to be nanosensitizers. ZnO, whose direct band gap is 3.37 eV [86], is one of the typical metallic oxide semiconductors applied in RT. The radioenhancing effect of ZnO NPs has been proven [87]. To evaluate the sensitizer effect of ZnO nanorods, Zhang et al. designed transferrin receptor antibody-functionalized ZnO nanorods loaded with doxorubicin for the concurrent chemoradiotherapy of hepatocellular carcinoma [47]. They used low-dose x-rays to irradiate SMMC-7721 cells within ZnO nanorods and observed an apparent decline in cell viability, increase in apoptotic cells in vitro and inhibition of tumor growth in vivo. Then, the researchers introduced Fe3O4 because its magnetic property allows visual MRI evaluation [88]. Consequently, ZnO nanomaterials function as a promising sensitizer for RT.
TiO2 is a wide band gap semiconductor that exhibits high photoactivity for generating ROS. Youkhana et al. synthesized anatase TiO2 NPs and observed radio enhancement of up to 67% in HaCaT and DU145 cells treated with TiO2 NPs and megavoltage x-rays [89]. The modification of PAA on the surface of bare TiOx NPs (synthesized from anatase-type TiO2 NPs) (Figure 5A) can prevent aggregation under physiological conditions. Cells treated with PAA-TiOx NPs and x-ray radiation had over twice the •OH levels (Figure 5E) and showed a higher level of apoptosis (Figure 5D) than the control groups. Additionally, the researchers' results showed that PAA-TiOx NPs decreased MIAPaCa-2 cell survival in vitro (Figure 5B) and inhibited tumor growth in vivo (Figure 5C), which was of great significance in assisting RT in the treatment of pancreatic cancer [48]. To load anticancer drugs for synergistic therapy, Dai et al. introduced TiOx nanosheets, which increased the production of •OH, O2•- and H2O2 under x-ray radiation, exhibiting promising radiosensitivity in A549 cells [90].

(A) Schematic illustration of the synthesis of PAA-TiOx NPs from anatase TiO2 NPs. (B) Colony-forming assay showing the combined effects of x-ray radiation and PAA-TiOx NPs. (C) Changes in tumor size after each treatment. (D) Images of the induction of apoptosis (evaluated by TUNEL assay). (E) Detection of intracellular ROS production and mean fluorescence values after each treatment are shown.
NP: Nanoparticle; ROS: Reactive oxygen species.
Reproduced with permission from [48], licensed with CC BY 4.0.
Some semiconductor nanomaterials containing high-Z elements also show their potential in radiosensitization, among which alkaline earth metal tungstates are representative nanosensitizers [91]. Jo et al. confirmed the radiosensitization effect of CaWO4 NPs both in vitro (head and neck cancer cells) and in vivo (xenografts) [92]. Soon after, Wang et al. found that BaWO4 NPs also work as radiosensitizers, and they observed that compared with treatment with x-rays alone, incubation with PVP-coated CaWO4 and BaWO4 increased the level of intracellular 1O2 by 17 and 15% and the level of intracellular •OH by 9.8- and 6.4-fold, respectively, reflecting that the generation of 1O2 and •OH is a key factor in the radiosensitization of metal tungstates [93].
Combination of semiconductors & high-Z elements
As mentioned earlier, high-Z elements increase the deposition of x-ray energy. Hence, there is a tendency to combine semiconductors with high-Z elements to achieve a higher level of ROS generation in radiosensitization. Several combined approaches have been explored in recent years, such as the use of TiO2 nanomaterials doped with lanthanide elements [94,95], Au [96] and tungsten [97] and ZnO nanomaterials doped with lanthanide elements [49,98]. For example, Ghaemi et al. doped ZnO NPs with europium and Gd to improve restricted radiation absorption [98]. Although the viability of cells treated with europium/Gd-doped ZnO NPs was found to be 40% of the viability of those treated with undoped ZnO NPs, the radiation dose remained the same because of europium- and Gd-mediated radiation increases and ROS generation. Similarly, Townley et al. designed titania NPs doped with lanthanides to improve localized energy absorption, and the mechanism of ROS generation in the presence of TiO2 and x-rays was similar to that of photoexcitation procedures [94]. An obvious decline of up to 75% in cell proliferation showed the potential of this approach to improve the effect of RT.
In these semiconductor nanomaterials, the electron–hole pairs induced by x-rays assist in generating ROS. However, the band gaps of these materials are sometimes narrow, which leads to the recombination of electron–hole pairs and a decreased catalytic reaction [78]. To overcome this obstacle, researchers have developed a strategy involving heterojunctions that present a wider barrier to reduce the probability of electron–hole pair recombination [99]. The combination of this strategy with metals such as Au or palladium provides considerable quantities of e- under x-ray radiation and helps to form a Schottky barrier between the metal and semiconductor, leading to an increasing level of ROS [100,101]. Inspired by these discoveries, Wang et al. developed metal-semiconductor (Au-Bi2S3) heteronanostructure composites with a Schottky barrier to improve the efficacy of RT for hypoxic tumors (Figure 6A & B) [50]. Increased levels of radicals and decreased cell proliferation in HeLa cells were observed in vitro (Figure 6C). In vivo, the Au-Bi2S3 + near-infrared radiation + x-ray group showed amazing tumor growth inhibition, where the tumors were almost completely ablated (Figure 6D). BiOI is able to generate electron–hole pairs under x-ray radiation and contains high-Z elements such as Bi and iodine. Guo et al. designed BiOI@Bi2S3@BSA semiconductor heterojunction NPs, in which Bi2S3 was coated on the surface of BiOI to form a heterojunction structure, which reduced electron–hole recombination and further enhanced the efficiency of electron–hole pair generation, thereby improving the efficiency of cancer-killing in both BEL-7402 cells and a mouse tumor model [102].

(A) Schematic illustration of RT enhancement based on the use of Au-Bi2S3 HNSCs. (B) Mechanism that may explain the enhanced ROS generation under radiation based on the Schottky-type heterostructures: the transfer of e- between Au and Bi2S3 brings the accumulation of e- to the surface of Au, resulting in the deformation of the band structure between Bi2S3 and Au, thus forming a Schottky barrier. (C) Fluorescence images of HeLa cells and HUVECs after different treatments (blue: cell nucleus; green: free radical generation). (D) Tumor growth curves of mice after different treatments.
Au: Gold; HNSC: Heteronanostructure composite; HUVEC: Human umbilical vein endothelial cell; ROS: Reactive oxygen species; RT: Radiotherapy.
Reproduced with permission from [50], © American Chemical Society (2019).
Carbon- & silicon-based semiconductors
Graphene quantum dots (GQDs) are novel graphene-based quasi-zero-dimensional carbon nanomaterials that show excellent characteristics, such as low cytotoxicity, good chemical modification potential and satisfactory biocompatibility and photoluminescence properties [103]. GQDs are rich in oxygen-containing groups and can serve as e- providers. When GQDs are excited, they can generate a variety of ROS, such as 1O2 via e- transfer and •OH and O2•- derived from electron–hole pairs. Encouraged by these findings, Ruan et al. prepared highly oxidized GQDs and demonstrated their powerful radiation enhancement in colorectal carcinoma therapy via the induction of apoptosis [51]. As seen in Figure 7A & B, in the group treated with radiation and GQDs, GQDs were efficiently endocytosed by colorectal carcinoma cells, and cell proliferation was greatly suppressed as a result of the increasing generation of intracellular as well as mitochondrial ROS (Figure 7C), leading to destructive apoptosis (Figure 7D).

(A) Cell viability of SW620 and HCT116 cells after different treatments. (B) Representative confocal fluorescence images and merged images of colorectal carcinoma cells (green: cytoskeleton protein of actin; red: GQDs; blue: nucleus). (C) Cell ROS productivity. Intracellular ROS levels in SW620 and HCT116 cells after different treatments are shown on the left. Mitochondrial ROS levels in SW620 and HCT116 cells after different treatments are shown on the right. (D) Apoptosis analyses of SW620 and HCT116 cells after different treatments for 24 h.
GQD: Graphene quantum dot; ROS: Reactive oxygen species.
Reproduced with permission from [51], © American Chemical Society (2018).
Silicon (Si) semiconductor NPs are another promising radiosensitizer because of their benign biodegradability and ability to generate ROS under radiation [104,105]. Si-NPs of sizes <5 nm efficiently improve the level of 1O2 in glioma C6 cells and present the potential for RT enhancement [106]. In terms of modifications, Klein et al. found that amino silanized Si-NPs (NH2-SiNPs) markedly increase ROS generation compared with uncapped Si-NPs because the amino functionality provides positive surface charges in aqueous environments [107]. After treatment with x-rays and NH2-SiNPs, MCF-7 and 3T3 cells showed increases in ROS concentration of 1.8- and 1.2-fold, respectively. Consequently, silicon nanomaterials could act as significant radiosensitizers to assist in RT.
Double-edged sword of the ROS generation strategy in RT
Although induced ROS can cause cancer cell death and inhibit cancer development, ROS generated from apoptotic and necrotic cells may promote tumor progression and metastasis through multiple mechanisms (Figure 8). ROS derived from damaged cells or activated immune cells during RT can work together with other damage-associated molecular patterns to accelerate the recruitment of macrophages and lymphocytes, thereby inducing the generation of proinflammatory cytokines and growth factors. This process will ultimately result in a sustained inflammatory response and enhanced tumor growth and metastasis [108,109].
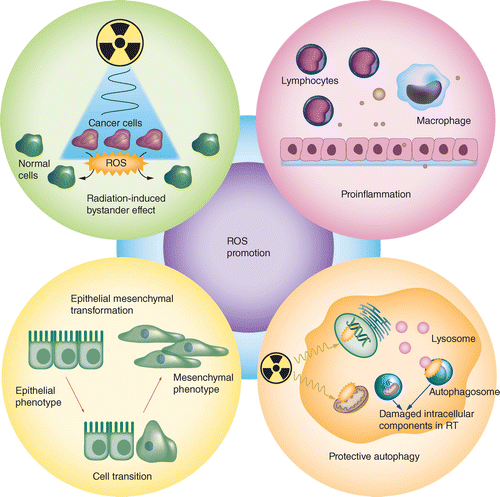
ROS: Reactive oxygen species; RT: Radiotherapy.
Nanosensitizer-induced ROS may damage normal cells via radiation-induced bystander effects (RIBEs), including increased levels of micronuclei, mutations and genomic instability, which can cause radiation-related secondary carcinogenesis. As small molecules, ROS can transmit bystander signals between adjacent cells through cell membrane or gap junction communication [110]. Elevated ROS can cause micronuclei in human hepatoma cells, implying the regulatory role of ROS in RIBEs [111], and the use of ROS scavengers effectively abolishes RIBEs induced by irradiated hepatoma cells [112]. Similarly, Zhang et al. used conditioned medium obtained after radiation to culture bone marrow-derived mesenchymal stem cells and observed the effect of RIBEs. They found that the RIBEs in these cells were mediated by ROS, which may be related to the MAPK/NF-κB signaling pathway [113].
Epithelial-to-mesenchymal transformation (EMT) endows cancer cells with greater migration and invasion abilities, which enhances radiation resistance [114]. ROS augment IR-induced EMT, and the use of ROS scavengers counteracts EMT induced by IR in breast cancer cells [115]. ROS induced by IR activate ERK, inactivate the downstream endogenous inhibitor of Snail (GSK3β) and ultimately increase the expression of Snail, a key inducible transcription factor in EMT [116]. Moreover, ROS induced by IR upregulate the expression of DLX-2, an upstream regulator of Snail in cancer cells, and promote the metastasis of cancer [117]. Furthermore, EMT and the production of ROS in IR seem to be important drivers of the development of cancer stem cells (CSCs), and CSCs show enhanced ROS insensitivity and lower ROS levels, leading to radioresistance [118,119]. The introduction of sensitizers may fail to produce enough ROS to kill CSCs. Li et al. investigated the effects of exogenous nanomaterials on ROS levels in liver cancer cells and liver CSCs and found that although the ROS level in ordinary cancer cells increased, exogenous nanomaterials at the same concentration showed a disappointing and insufficient ROS level in CSCs, which ultimately facilitates cell proliferation and survival [120].
Generally, there are two highly distinct effects of elevated autophagy in cancer therapy: prosurvival and prodeath [121]. Although several of the sensitizers discussed earlier enhance tumor-killing by inducing autophagy, it is still worth noting that protective autophagy activated by ROS could in turn protect tumor cells against ROS-induced damage and lead to unsatisfactory anticancer effects. For instance, after treatment with Ag NPs and radiation, ROS generated in tumor cells increase the formation of autophagosomes, and the blockage of protective autophagy improves the effectiveness of RT [43]. In breast cancer, ROS activate TFE3, which upregulates the expression of LC3 and LAMP-1 mRNA, consequently increasing autophagy [122]. In lung adenocarcinoma cells, ROS derived from low-dose IR induce radioresistance by promoting the autophagy process, and ROS scavengers attenuate the IR-induced activation of autophagy [123]. Therefore, the double-edged sword of the effects of ROS on autophagy should be fully recognized to balance the relationship between the induction and inhibition of autophagy.
It is still rare to see reports that ROS derived from sensitizers promote tumor recurrence and metastasis and increase radiation resistance. Most of the existing studies on this topic have focused on the short-term tumor-killing effect, and few studies have investigated whether RT nanosensitizers induce unexpected outcomes in the long term. However, because elevated ROS levels during RT ultimately influence the effectiveness of treatment, the hidden dangers of sensitizers with an ROS-promoting ability cannot be ignored.
Conclusion
Promoting ROS generation is a promising strategy in nanosensitizer-mediated radiotherapy. The principles of catalyzing ROS production can be divided into three categories. First, high-Z nanosensitizers enhance the photoelectric effect and increase the emission of secondary electrons. Second, transition metal-based nanosensitizers catalyze the Haber–Weiss and Fenton reactions. Third, semiconducting nanosensitizers form highly reactive electron–hole pairs under x-ray excitation. However, the hidden dangers of nanosensitizers with ROS-promoting ability should not be ignored and need to be further explored.
Future perspective
Emerging nanomaterials provide a novel idea for improving the efficiency of RT, and promoting ROS generation is a promising strategy in nanosensitizer-mediated tumor radiotherapy. Excessive ROS inhibit tumor growth by directly attacking biological macromolecules, destroying the cell structure and inducing various forms of cell death. Nanosensitizers promote the formation of ROS through a wide range of effects, such as enhancing the photoelectric effect, catalyzing the Haber–Weiss and Fenton reactions and forming electron–hole pairs, all of which enhance radiosensitivity. However, a variety of challenges still need to be addressed:
Optimization of the properties of nanosensitizers. Size, shape, chemical structure and surface functional groups can all determine ROS generation efficacy, so nanosensitizer preparation and IR therapeutic efficiency should receive more attention. Moreover, therapeutic efficiency is related to the effective concentration and time of application, and insufficient or excessive amounts of a sensitizer negatively influence tumor RT. To improve therapeutic efficiency of IR, it is important to develop and optimize sensitizers with good biocompatibility, a high ROS quantum yield and accurate targeting effects;
Concrete effect mechanism of nanosensitizers. To develop an effective nanosensitizer for further clinical application, the concrete effect mechanism of sensitizers on tumor RT should be clarified. There is little research showing how nanosensitizer-induced ROS are involved in regulating cancer cell growth, migration and invasion. Therefore, it is necessary to investigate the molecular pathway of sensitizer-induced, ROS-regulated cell death in RT, rather than merely demonstrating the efficacy of the sensitizer, as shown in current studies. Only when the detailed downstream pathway of tumor suppression is fully elucidated will it be possible to avoid negative sensitizer-induced effects. Moreover, the term ‘ROS’ does not refer to a single molecule but to a series of molecular oxygen derivatives, which may present different functions and mechanisms in radiosensitization. However, individual ROS compounds are rarely distinguished in the current research. With the development of chemical detection and imaging techniques, it is necessary to study the radiosensitization effect of specific ROS molecules in the future to better explore the concrete molecular mechanisms;
Monitoring and control of ROS levels. The biological effects of ROS are confusing. ROS derived from sensitizers in RT not only kill the tumor but also present hidden dangers regarding tumor recurrence. Therefore, it is particularly important to monitor and control the production of ROS to achieve satisfactory therapeutic efficacy and avoid potential cancer-promoting effects. Moreover, it has been recognized that the cytotoxicity threshold of ROS in CSCs is higher than that of tumor cells. Therefore, management of ROS to achieve an appropriate concentration at which CSCs can also be inhibited by RT will be a challenging topic for overcoming tumor recurrence and radioresistance.
Nanomaterials achieve a surprising radiosensitization effect through the production of ROS. Unfortunately, the clinical translation of nanosensitizers is still difficult because of the aforementioned problems. However, we believe that with the settlement of these issues in a step-by-step manner, sensitizers will certainly give rise to new possibilities for RT, effectively reducing the risk of high-dose radiation and preventing tumor recurrence and metastasis after RT.
Background
Radiotherapy (RT) is one of the main clinical treatments for many types of cancer, but high-dose radiation damages healthy tissues and causes serious side effects.
Introducing nanosensitizers is a promising method for reducing RT-related side effects.
Catalyzing reactive oxygen species (ROS) generation is a key strategy in nanosensitizer-mediated RT.
ROS are broadly described as chemical species derived from molecular oxygen, and numerous therapeutic strategies based on ROS cytotoxicity have been proven effective for inhibiting tumor growth.
Mechanism of ROS-induced cancer cell death
In RT, radiation interacts with water molecules to form H2O+ and a free electron. These products then react with another water molecule to form highly active •OH or other ROS species.
ROS directly damage biomolecules, such as lipids, proteins and DNA.
As a second messenger, ROS trigger multiple cell death through a variety of mechanisms, including apoptosis, autophagy, necroptosis, ferroptosis and pyroptosis.
Nanosensitizers facilitate ROS generation via three different principles
High-Z element-based nanosensitizers facilitate ROS generation by enhancing the photoelectric effect. For example, nanosensitizers based on gold (Z = 79), silver (Z = 47), cerium (Z = 58), gadolinium (Z = 64), hafnium (Z = 72), tungsten (Z = 74), iridium (Z = 77), platinum (Z = 78) and bismuth (Z = 83).
Transition metal-based nanosensitizers facilitate ROS generation via the catalysis of the Haber–Weiss and Fenton reactions. For example, iron- and copper-based nanosensitizers.
Semiconducting sensitizers facilitate ROS generation via the formation of electron–hole pairs. For example, metal-based semiconductors, combination of semiconductor and high-Z elements and carbon- and silicon-based semiconductors.
Hidden dangers of nanosensitizers with an ROS-promoting ability
ROS derived from damaged cells or activated immune cells during RT result in a sustained inflammatory response and enhanced tumor growth and metastasis.
Nanosensitizer-induced ROS may damage normal cells via radiation-induced bystander effects and lead to secondary carcinogenesis.
ROS augment ionizing radiation-induced epithelial-to-mesenchymal transformation, which endows cancer cells with greater migration and invasion abilities.
ROS may activate protective autophagy, which protects tumor cells against ROS-induced damage, and lead to unsatisfactory anticancer effects.
A variety of challenges still need to be addressed
It is necessary to develop and optimize sensitizers with good biocompatibility, high ROS quantum yield and accurate targeting effects.
It is necessary to investigate the molecular pathway of sensitizer-induced, ROS-regulated cell death in RT and study the radiosensitization effect of specific ROS molecules.
It is necessary to monitor and control the production of ROS to achieve satisfactory therapeutic efficacy and avoid potential cancer-promoting effects.
Financial & competing interests disclosure
This work was supported by the National Natural Science Foundation of China (no. 81972530 and U1932135); the Fund for Excellent Young Scholars of Shanghai Ninth People's Hospital, Shanghai Jiao Tong University School of Medicine (no. JYYQ001); the National Key Research and Development Program of China (no. 2018YFC1106100 and 2018YFC1106101); the Shanghai Rising-Star Program (no. 17QA1402000); the scholarship from the China Scholarship Council (no. 201906235030); and the research grant from the Shanghai Science and Technology Committee (no. 17DZ2260100). The authors have no other relevant affiliations or financial involvement with any organization or entity with a financial interest in or financial conflict with the subject matter or materials discussed in the manuscript apart from those disclosed.
No writing assistance was utilized in the production of this manuscript.
Open access
This work is licensed under the Attribution-NonCommercial-NoDerivatives 4.0 Unported License. To view a copy of this license, visit http://creativecommons.org/licenses/by-nc-nd/4.0/
Papers of special note have been highlighted as: • of interest; •• of considerable interest
References
- 1. . Development of clinical radiotherapy since 1896. Acta Oncol. 34(8), 995–1003 (1995).
- 2. . Recent developments in radiotherapy. N. Engl. J. Med. 377(11), 1065–1075 (2017).
- 3. Metal-based nanoenhancers for future radiotherapy: radiosensitizing and synergistic effects on tumor cells. Theranostics 8(7), 1824–1849 (2018).
- 4. . Therapeutic radiometals beyond (177)Lu and (90)Y: production and application of promising alpha-particle, beta(−)-particle, and Auger electron emitters. J. Nucl. Med. 58(Suppl. 2), 91S–96S (2017).
- 5. Preoperative or postoperative radiotherapy versus surgery alone for retroperitoneal sarcoma: a case–control, propensity score-matched analysis of a nationwide clinical oncology database. Lancet Oncol. 17(7), 966–975 (2016).
- 6. . Palliative radiotherapy. BMJ 360, k821 (2018).
- 7. . Radiotherapy toxicity. Nat. Rev. Dis. Primers 5(1), 13 (2019).
- 8. Modifications in glass ionomer cements: nano-sized fillers and bioactive nanoceramics. Int. J. Mol. Sci. 17(7), 1134 (2016).
- 9. . The potential effectiveness of nanoparticles as radio sensitizers for radiotherapy. Bioimpacts 4(1), 15–20 (2014).
- 10. . Nanomaterial-based blood–brain-barrier (BBB) crossing strategies. Biomaterials 224, 119491 (2019).
- 11. . Nanomedicine-based immunotherapy for the treatment of cancer metastasis. Adv. Mater. 31(49), e1904156 (2019).
- 12. . Emerging strategies of nanomaterial-mediated tumor radiosensitization. Adv. Mater. 31(3), e1802244 (2019).
- 13. . Targeting antioxidant enzymes as a radiosensitizing strategy. Cancer Lett. 438, 154–164 (2018). • Shows the importance of reactive oxygen species (ROS) in radioresponse.
- 14. . Reactive oxygen species (ROS) as pleiotropic physiological signalling agents. Nat. Rev. Mol. Cell Biol. 21(7), 363–383 (2020). •• Thoroughly describes the concept of the term ‘ROS’ and the specific species.
- 15. The interplay of reactive oxygen species, hypoxia, inflammation, and sirtuins in cancer initiation and progression. Oxid. Med. Cell. Longev. 2016, 3907147 (2016).
- 16. . Induction of reactive oxygen species: an emerging approach for cancer therapy. Apoptosis 22(11), 1321–1335 (2017).
- 17. . Photosensitized oxidation of membrane lipids: reaction pathways, cytotoxic effects, and cytoprotective mechanisms. J. Photochem. Photobiol. B 63(1–3), 103–113 (2001).
- 18. . Oxidation events and skin aging. Ageing Res. Rev. 21, 16–29 (2015).
- 19. . Mitochondrial reactive oxygen species (ROS) and ROS-induced ROS release. Physiol Rev. 94(3), 909–950 (2014).
- 20. The Fas death signaling pathway connecting reactive oxygen species generation and FLICE inhibitory protein down-regulation. J. Immunol. 180(5), 3072–3080 (2008).
- 21. Cytochrome c acts as a cardiolipin oxygenase required for release of proapoptotic factors. Nat. Chem. Biol. 1(4), 223–232 (2005).
- 22. Ochratoxin A-induced apoptosis of IPEC-J2 cells through ROS-mediated mitochondrial permeability transition pore opening pathway. J. Agric. Food Chem. 65(48), 10630–10637 (2017).
- 23. Oxidative modification of caspase-9 facilitates its activation via disulfide-mediated interaction with Apaf-1. Cell Res. 19(4), 449–457 (2009).
- 24. . Reactive oxygen species (ROS) control the expression of Bcl-2 family proteins by regulating their phosphorylation and ubiquitination. Cancer Sci. 95(8), 644–650 (2004).
- 25. . Biological functions of autophagy genes: a disease perspective. Cell 176(1–2), 11–42 (2019).
- 26. . mTOR: a pharmacologic target for autophagy regulation. J. Clin. Invest. 125(1), 25–32 (2015).
- 27. Adrenomedullin alleviates the pyroptosis of Leydig cells by promoting autophagy via the ROS-AMPK-mTOR axis. Cell Death Dis. 10(7), 489 (2019).
- 28. Delicaflavone induces ROS-mediated apoptosis and inhibits PI3K/AKT/mTOR and Ras/MEK/Erk signaling pathways in colorectal cancer cells. Biochem. Pharmacol. 171, 113680 (2020).
- 29. . Nuclear receptor 4A1 (NR4A1) antagonists induce ROS-dependent inhibition of mTOR signaling in endometrial cancer. Gynecol Oncol. 154(1), 218–227 (2019).
- 30. . Reactive oxygen species are essential for autophagy and specifically regulate the activity of Atg4. EMBO J. 26(7), 1749–1760 (2007).
- 31. . Oxidative stress induces autophagic cell death independent of apoptosis in transformed and cancer cells. Cell Death Differ. 15(1), 171–182 (2008).
- 32. The role of necroptosis in ROS-mediated cancer therapies and its promising applications. Cancers (Basel) 12(8), 2185 (2020).
- 33. RIP1 autophosphorylation is promoted by mitochondrial ROS and is essential for RIP3 recruitment into necrosome. Nat. Commun. 8, 14329 (2017).
- 34. Shikonin induces glioma cell necroptosis in vitro by ROS overproduction and promoting RIP1/RIP3 necrosome formation. Acta Pharmacol. Sin. 38(11), 1543–1553 (2017).
- 35. . Recent progress in ferroptosis inducers for cancer therapy. Adv. Mater. 31(51), e1904197 (2019).
- 36. The role of ferroptosis in ionizing radiation-induced cell death and tumor suppression. Cell Res. 30(2), 146–162 (2020).
- 37. . Caspase-1-induced pyroptotic cell death. Immunol. Rev. 243(1), 206–214 (2011).
- 38. Nicotine promotes atherosclerosis via ROS-NLRP3-mediated endothelial cell pyroptosis. Cell Death Dis. 9(2), 171 (2018).
- 39. Mitochondrial ROS promote macrophage pyroptosis by inducing GSDMD oxidation. J. Mol. Cell Biol. 11(12), 1069–1082 (2019).
- 40. Oxeiptosis, a ROS-induced caspase-independent apoptosis-like cell-death pathway. Nat. Immunol. 19(2), 130–140 (2018).
- 41. . Enhancing the radiotherapeutic index of gamma radiation on cervical cancer cells by gold nanoparticles. Gold Bull. 52(3–4), 185–196 (2019).
- 42. Atomically precise gold-levonorgestrel nanocluster as a radiosensitizer for enhanced cancer therapy. ACS Nano 13(7), 8320–8328 (2019).
- 43. Reactive oxygen species acts as executor in radiation enhancement and autophagy inducing by AgNPs. Biomaterials 101, 1–9 (2016).
- 44. Radiosensitizing effect of gadolinium oxide nanocrystals in NSCLC cells under carbon ion irradiation. Nanoscale Res. Lett. 14(1), 328 (2019).
- 45. Copper oxide nanoparticles induce enhanced radiosensitizing effect via destructive autophagy. ACS Biomater. Sci. Eng. 5(3), 1569–1579 (2019).
- 46. . Targeted iron oxide nanoparticles for the enhancement of radiation therapy. Biomaterials 105, 127–135 (2016).
- 47. . Targeting and noninvasive treatment of hepatocellular carcinoma in situ by ZnO nanorod-mediated concurrent chemoradiotherapy. RSC Adv. 5(104), 85720–85729 (2015).
- 48. Titanium peroxide nanoparticles enhanced cytotoxic effects of x-ray irradiation against pancreatic cancer model through reactive oxygen species generation in vitro and in vivo. Radiat. Oncol. 11(1), 91 (2016).
- 49. . Enhanced cytotoxic and genotoxic effects of gadolinium-doped ZnO nanoparticles on irradiated lung cancer cells at megavoltage radiation energies. Mater. Sci. Eng. C Mater. Biol. Appl. 103, 109739 (2019).
- 50. Enhanced generation of non-oxygen dependent free radicals by Schottky-type heterostructures of Au-Bi2S3 nanoparticles via x-ray-induced catalytic reaction for radiosensitization. ACS Nano 13(5), 5947–5958 (2019). •• Developed a Schottky-type heterostructure of Au–Bi2S3 with promising ability of ROS generation via efficient separation of the electron−hole pairs.
- 51. Graphene quantum dots for radiotherapy. ACS Appl. Mater. Interfaces 10(17), 14342–14355 (2018).
- 52. Size-dependent radiosensitization of PEG-coated gold nanoparticles for cancer radiation therapy. Biomaterials 33(27), 6408–6419 (2012).
- 53. Enhanced tumor accumulation of sub-2 nm gold nanoclusters for cancer radiation therapy. Adv. Healthc. Mater. 3(1), 133–141 (2014).
- 54. . Gold nanoparticles for radiosensitizing and imaging of cancer cells. Radiat. Phys. Chem. 152, 137–144 (2018).
- 55. Labeling monocytes with gold nanoparticles to track their recruitment in atherosclerosis with computed tomography. Biomaterials 87, 93–103 (2016).
- 56. . Advances and potential application of gold nanoparticles in nanomedicine. J. Cell. Biochem. 120(10), 16370–16378 (2019).
- 57. Shape-dependent radiosensitization effect of gold nanostructures in cancer radiotherapy: comparison of gold nanoparticles, nanospikes, and nanorods. ACS Appl. Mater. Interfaces 9(15), 13037–13048 (2017).
- 58. . Action of gold nanospikes-based nanoradiosensitizers: cellular internalization, radiotherapy, and autophagy. ACS Appl. Mater. Interfaces 9(37), 31526–31542 (2017).
- 59. Thio-glucose bound gold nanoparticles enhance radio-cytotoxic targeting of ovarian cancer. Nanotechnology 22(28), 285101 (2011).
- 60. . A gold nanoparticle system for the enhancement of radiotherapy and simultaneous monitoring of reactive-oxygen-species formation. Nanotechnology 29(50), 504001 (2018).
- 61. . Large-scale synthesis of flexible free-standing SERS substrates with high sensitivity: electrospun PVA nanofibers embedded with controlled alignment of silver nanoparticles. ACS Nano 3(12), 3993–4002 (2009).
- 62. . Silver nanoparticles coupled to anti-EGFR antibodies sensitize nasopharyngeal carcinoma cells to irradiation. Mol. Med. Rep. 16(6), 9005–9010 (2017).
- 63. . Synergetic effect of silver nanoparticles and UVC irradiation on H2AX gene expression in TK6 cells. Cell J. 21(2), 204–209 (2019).
- 64. X-ray-controlled generation of peroxynitrite based on nanosized LiLuF4:Ce3+ scintillators and their applications for radiosensitization. Adv. Mater. 30(43), e1804046 (2018).
- 65. . Co-delivery of PARP and PI3K inhibitors by nanoscale metal-organic frameworks for enhanced tumor chemoradiation. Nano Res. 12(12), 3003–3017 (2019).
- 66. Hafnium oxide as a nanoradiosensitizer under x-ray irradiation of aqueous organic systems: a model study using the spin-trapping technique and Monte Carlo simulations. J. Phys. Chem. C 123(45), 27375–27384 (2019).
- 67. Ultrasmall biocompatible WO3- x nanodots for multi-modality imaging and combined therapy of cancers. Adv. Mater. 28(25), 5072–5079 (2016).
- 68. Iridium nanocrystals encapsulated liposomes as near-infrared light controllable nanozymes for enhanced cancer radiotherapy. Biomaterials 181, 81–91 (2018).
- 69. . Enhanced melanoma cell-killing by combined phototherapy/radiotherapy using a mesoporous platinum nanostructure. Photodiagn. Photodyn. Ther. 28, 300–307 (2019).
- 70. Facile synthesis of Bi2S3-MoS2 heterogeneous nanoagent as dual functional radiosensitizer for triple negative breast cancer theranostics. Chem. Eng. J. 395, 125032 (2020).
- 71. Suppressing the radiation-induced corrosion of bismuth nanoparticles for enhanced synergistic cancer radio-phototherapy. ACS Nano 14(10), 13016–13029 (2020). • Creatively designed artificial heterostructures with graphene nanosheets to greatly suppress radiation-induced corrosion in bismuth nanoparticles and decrease depletion of ROS.
- 72. Enhanced cisplatin chemotherapy by iron oxide nanocarrier-mediated generation of highly toxic reactive oxygen species. Nano Lett. 17(2), 928–937 (2017).
- 73. . Superparamagnetic iron oxide nanoparticles as radiosensitizer via enhanced reactive oxygen species formation. Biochem. Biophys. Res. Commun. 425(2), 393–397 (2012).
- 74. Enhanced in vitro biocompatibility and water dispersibility of magnetite and cobalt ferrite nanoparticles employed as ROS formation enhancer in radiation cancer therapy. Small 14(21), e1704111 (2018). •• Self-assembled monolayer-stabilized Fe3O4 and CoFe2O4 nanoparticles increased level of ROS in MCF-7 breast cancer cells but not in healthy cells, exhibiting their excellent applicability in radiotherapy.
- 75. . Multifunctional nanocomposites MGO/FU-MI inhibit the proliferation of tumor cells and enhance the effect of chemoradiotherapy in vivo and in vitro. Clin. Transl. Oncol. 22(10), 1875–1884 (2020).
- 76. . Understanding the pathway of antibacterial activity of copper oxide nanoparticles. RSC Adv. 5(16), 12293–12299 (2015).
- 77. Design of TPGS-functionalized Cu3BiS3 nanocrystals with strong absorption in the second near-infrared window for radiation therapy enhancement. Nanoscale 9(24), 8229–8239 (2017).
- 78. . Photocatalytic and radiocatalytic nanomaterials for the degradation of organic species. Radiat. Phys. Chem. 84, 51–58 (2013).
- 79. . ZnO nanoparticles: a promising anticancer agent. Nanobiomedicine (Rij) 3, 9 (2016).
- 80. . Treatment of organic pollutants in water using TiO2 powders: photocatalysis versus sonocatalysis. React. Kinet. Mech. Catal. 109(2), 335–354 (2013).
- 81. . Nanoparticles of titanium and zinc oxides as novel agents in tumor treatment: a review. Nanoscale Res. Lett. 12, 225 (2017).
- 82. . The present and future role of photodynamic therapy in cancer treatment. Lancet Oncol. 5(8), 497–508 (2004).
- 83. Marriage of scintillator and semiconductor for synchronous radiotherapy and deep photodynamic therapy with diminished oxygen dependence. Angew. Chem. Int. Ed. Engl. 54(6), 1770–1774 (2015).
- 84. . Mn-doped ZnS quantum dots-chlorin e6 shows potential as a treatment for chondrosarcoma: an in vitro study. IET Nanobiotechnol. 13(4), 387–391 (2019).
- 85. Tungsten sulfide quantum dots as multifunctional nanotheranostics for in vivo dual-modal image-guided photothermal/radiotherapy synergistic therapy. ACS Nano 9(12), 12451–12463 (2015).
- 86. . Synthesis and characterization of ZnO nanowires and ZnO-CuO nanoflakes from sputter-deposited brass (Cu-0.65-Zn-0.35) film and their application in gas sensing. J. Mater. Sci. Technol. 31(11), 1069–1078 (2015).
- 87. . Radiosensitizing effect of zinc oxide and silica nanocomposites on cancer cells. Colloids Surf. B Biointerfaces 129, 79–86 (2015).
- 88. . Theranostics for hepatocellular carcinoma with Fe3O4@ZnO nanocomposites. Biomater Sci. 4(2), 288–298 (2016).
- 89. . Titanium dioxide nanoparticles as radiosensitisers: an in vitro and phantom-based study. Int. J. Med. Sci. 14(6), 602–614 (2017).
- 90. Dual-stimuli-responsive TiOx/DOX nanodrug system for lung cancer synergistic therapy. RSC Adv. 8(39), 21975–21984 (2018).
- 91. . Bilirubin-coated radioluminescent particles for radiation-induced photodynamic therapy. ACS Appl. Bio Mater. 3(8), 4858–4872 (2020).
- 92. PEG-PLA-coated and uncoated radio-luminescent CaWO4 micro- and nanoparticles for concomitant radiation and UV-A/radio-enhancement cancer treatments. ACS Biomater. Sci. Eng. 4(4), 1445–1462 (2018).
- 93. Barium tungstate nanoparticles to enhance radiation therapy against cancer. Nanomedicine 28, 102230 (2020).
- 94. . Nanoparticle augmented radiation treatment decreases cancer cell proliferation. Nanomedicine 8(4), 526–536 (2012).
- 95. Enhancement of mitochondrial ROS accumulation and radiotherapeutic efficacy using a Gd-doped titania nanosensitizer. Theranostics 9(1), 167–178 (2019).
- 96. . X-ray radiation enhancement of gold- TiO2 nanocomposites. Appl. Surface Sci. 480, 1147–1155 (2019).
- 97. W-doped TiO2 nanoparticles with strong absorption in the NIR-II window for photoacoustic/CT dual-modal imaging and synergistic thermoradiotherapy of tumors. Theranostics 9(18), 5214–5226 (2019).
- 98. . Harnessing the cancer radiation therapy by lanthanide-doped zinc oxide based theranostic nanoparticles. ACS Appl. Mater. Interfaces 8(5), 3123–3134 (2016).
- 99. Semiconductor heterojunction photocatalysts: design, construction, and photocatalytic performances. Chem. Soc. Rev. 43(15), 5234–5244 (2014).
- 100. . Palladium-based nanomaterials: a platform to produce reactive oxygen species for catalyzing oxidation reactions. Adv. Mater. 27(44), 7025–7042 (2015).
- 101. Chemical design of palladium-based nanoarchitectures for catalytic applications. Small 15(6), e1804378 (2019).
- 102. Synthesis of BSA-coated BiOI@Bi2 S3 semiconductor heterojunction nanoparticles and their applications for radio/photodynamic/photothermal synergistic therapy of tumor. Adv. Mater. 29(44), (2017).
- 103. . Graphene quantum dots for optical bioimaging. Small 15(36), e1902136 (2019).
- 104. . Nanodiamonds and silicon quantum dots: ultrastable and biocompatible luminescent nanoprobes for long-term bioimaging. Chem. Soc. Rev. 44(14), 4853–4921 (2015).
- 105. . Two-photon excitation nanoparticles for photodynamic therapy. Chem. Soc. Rev. 45(24), 6725–6741 (2016).
- 106. ROS enhancement by silicon nanoparticles in x-ray irradiated aqueous suspensions and in glioma C6 cells. J. Nanoparticle Res. 14(3), 741 (2012).
- 107. Oxidized silicon nanoparticles for radiosensitization of cancer and tissue cells. Biochem. Biophys. Res. Commun. 434(2), 217–222 (2013).
- 108. . Key role of DAMP in inflammation, cancer, and tissue repair. Clin. Ther. 38(5), 1017–1028 (2016).
- 109. . Immunological markers that predict radiation toxicity. Cancer Lett. 368(2), 191–197 (2015).
- 110. . Radiation-induced bystander and systemic effects serve as a unifying model system for genotoxic stress responses. Mutat. Res. 778, 13–22 (2018).
- 111. Role of ROS-mediated autophagy in radiation-induced bystander effect of hepatoma cells. Int. J. Radiat. Biol. 91(5), 452–458 (2015).
- 112. SirT1 knockdown potentiates radiation-induced bystander effect through promoting c-Myc activity and thus facilitating ROS accumulation. Mutat. Res. 772, 23–29 (2015).
- 113. Astragalus polysaccharide inhibits ionizing radiation-induced bystander effects by regulating MAPK/NF-kB signaling pathway in bone mesenchymal stem cells (BMSCs). Med. Sci. Monit. 24, 4649–4658 (2018).
- 114. . New insights into the mechanisms of epithelial–mesenchymal transition and implications for cancer. Nat. Rev. Mol. Cell Biol. 20(2), 69–84 (2019).
- 115. . Effects of N-acetyl-L-cysteine on adhesive strength between breast cancer cell and extracellular matrix proteins after ionizing radiation. Life Sci. 93(21), 798–803 (2013).
- 116. . ERK/GSK3beta/Snail signaling mediates radiation-induced alveolar epithelial-to-mesenchymal transition. Free Radic. Biol. Med. 52(6), 983–992 (2012).
- 117. Reactive oxygen species induce epithelial-mesenchymal transition, glycolytic switch, and mitochondrial repression through the Dlx-2/Snail signaling pathways in MCF-7 cells. Mol. Med. Rep. 20(3), 2339–2346 (2019).
- 118. . Cancer stem cell (CSC) resistance drivers. Life Sci. 234, 116781 (2019).
- 119. Induction of metastasis, cancer stem cell phenotype, and oncogenic metabolism in cancer cells by ionizing radiation. Mol. Cancer 16(1), 10 (2017).
- 120. Effects of quantum dots on the ROS amount of liver cancer stem cells. Colloids Surf. B Biointerfaces 155, 193–199 (2017).
- 121. . Autophagy in cell death: an innocent convict? J. Clin. Invest. 115(10), 2679–2688 (2005).
- 122. Inhibiting ROS-TFE3-dependent autophagy enhances the therapeutic response to metformin in breast cancer. Free Radic. Res. 52(8), 872–886 (2018).
- 123. . ROS/autophagy/NRF2 pathway mediated low-dose radiation induced radio-resistance in human lung adenocarcinoma A549 cell. Int. J. Biol. Sci. 11(7), 833–844 (2015).