Methods for powering bioelectronic microdevices
Abstract
Bioelectronic microdevices are an emerging class of biomedical devices miniaturized at the scale of a millimeter or less, which promise new capabilities for monitoring and treating human disease. Although rapid progress has been made in the sensing and actuation capabilities of microdevices, a major technological challenge remains in the way that these devices are powered within the body. In this review, we revisit the power requirements of microdevices, describe current methods for storing, transferring or harvesting energy in microdevices, provide an overview of emerging powering approaches and discuss the promise of microdevices in biomedicine.
Beginning with the implantation of the pacemaker in the early 1960s [1], the miniaturization of electronics has enabled new approaches to diagnose and treat human diseases. Notable examples in modern medicine include cochlear implants for restoring hearing [2], ingestible endoscopes for imaging the GI tract [3,4] and sensors for brain interfacing [5,6]. The continued miniaturization of bioelectronics from the current scale of centimeters to the microscale promises to similarly expand the role of bioelectronics in medicine. In particular, devices capable of functioning autonomously on the scale of a single millimeter or less, termed here ‘microdevices’, could provide unique capabilities unavailable with existing technologies, such as precise access to physiological structures in the body, compatibility with minimally invasive implantation techniques and evasion of the body's foreign body response [7]. The ability of such microdevices to integrate with the body and target precise regions could be key to realizing major goals in biomedicine, including the large-scale mapping of brain function [8], therapeutic control of organ functions by peripheral nerve modulation [9] and localized drug delivery with spatial and temporal accuracy [10].
Powering represents the most pressing technological challenge hindering the realization and clinical use of bioelectronic microdevices. Although recent advances in micro- and nanotechnology have enabled the fabrication of microdevices with sophisticated sensing, actuation and signal-processing capabilities [11–15], their ability to reliably function in the body remains constrained by limitations of existing powering technology, which generally involve centimeter-scale architectures. The development of new methods to transfer, harvest and store energy in the complex and dynamic environment of the human body is required to enable the deployment of microdevices in the human body.
In this review, we provide an overview of current and emerging methods for powering microdevices. We first summarize the power budgets required for state-of-the-art bioelectronics, then describe current strategies for storing, transferring or harvesting storing energy in microdevices (Figure 1) by outlining the principles of operation, performance and specific application examples. We next discuss emerging methods for powering microdevices, focusing on their current limitations and potential approaches for addressing them. Finally, we conclude with an outlook on prospective routes to efficient powering of microdevices.
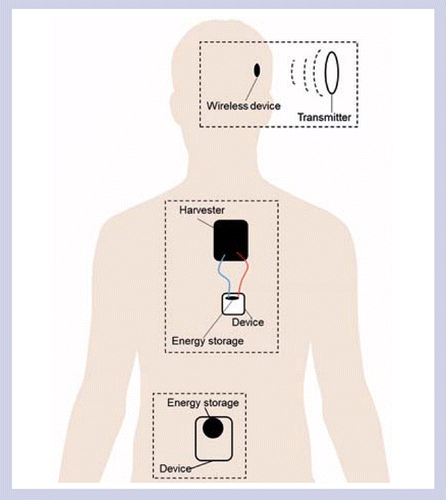
Methods may be categorized by whether they store, harvest or transfer energy to the device. Microdevices can currently be powered by energy transfer methods in certain physiological regions and by energy storage methods over short durations. Energy harvesting methods require improved performance in order to be compatible with a microdevice.
Power requirements of bioelectronic microdevices
The electronics of a microdevice consists of various functional blocks for sensing, signal processing, communication, actuation and power management. The energy consumption of each block can vary by many orders of magnitude, ranging from 1 μW to 10 mW, depending on the constraints set by the application. We briefly review the typical power budget for different functional blocks in a microdevice for basic design guidance. State-of-the-art techniques in low power circuits and architectures can provide significant gains beyond these typical requirements [16]. Continued advances in the design with low-power functional blocks will reduce the both operational and standby power consumption, which combined with powering techniques can prolong the operational lifetime of bioelectronics and enable their practical applications in the foreseeable future.
Sensing
Sensing devices convert physiological activity to electrical signals. Typically, sensing consumes the least power (<10 μW) compared with other functions such as actuation or communication [17–20]. Sensors can be entirely passive, such as capacitive pressure sensors used for applications such as intraocular pressure monitoring [21]. For these devices, the energy consumption is dominated by the blocks for communication or, if necessary, analog-to-digital conversion. Active sensors incorporate additional blocks for signal amplification and processing. A prominent example is neural recording, which consumes about 5 μW per channel [22], may involve a large number of channels (1–10 mW, up to thousands of channels) [23,24].
Electrical stimulation
The therapeutic function of many bioelectronic devices (such as pacemakers and cochlear implants) is based on the modulation of electrical activity. The amount of power needed to activate neural activity depends on the excitability of the tissue, the impedance of the electrodes (which increases with time due to biofouling) [25–27], the duty cycle of the stimulation protocol and efficiency of the circuitry. The activation of impulses on nerves requires approximately 10–100 μW of power [28,29], while muscle stimulation typically involves 1–10 mW owing to higher activation thresholds [30,31]. Modern implantable pacemakers and defibrillators require only 5–10 μW, enabling about 10 years of operation on a single battery [32,33]. More recent applications such as stimulation of retinal prosthesis chip consume about 10–100 μW per electrode [34,35].
Communication
Wireless communication with devices external to the body is critical for the transmission of sensor data or control instructions. Active transmission of data using radiofrequency systems generally requires from 10 μW to 10 mW [36,37], depending on the distance from the receiver and the depth of the device in the body. Alternative methods based on acoustic waves or electric potentials require similar orders of magnitude of power. Because of the relatively high power requirements, devices usually communicate infrequently in order to reduce energy consumption. Alternatively, passive approaches based on modulation of the signal scattered by the device can be used to communicate without directly consuming power. However, such methods also constrain the design of the device, in particular requiring antennas with large dimensions to generate detectable scattering. Because passive communication methods rely on the absorption and re-emission of incident energy, they are subject to the same physical limitations inherent to energy transfer (see section on energy transfer).
Other functions
Bioelectronics may also modulate physiological activity in nonelectrical ways. For instance, light delivery-using implantable light-emitting diodes have been used for optogenetics and photodynamic therapy [37]; typically 2–10 mW is required for effective activation of the molecular constructs. Electrothermal microactuators used to move electrodes and monitor electrical activity of single neurons in the brain reported a power consumption of 91 mW [38]. For drug release, ignition of a microthruster to expel drugs from a reservoir required 166 mW [39].
Current methods for powering microdevices
Most medical devices today are powered by batteries, which have been used to power pacemakers since 1960s and continue to be widely used due to their safety and reliability. However, technological limitations in energy storage performance currently necessitate batteries with centimeter-scale dimensions in order to power bioelectronic devices over long durations. Most demonstrations of microdevices so far circumvent these limitations by using energy transfer techniques to deliver power to the device through the biological tissue. Microdevices less than a single millimeter in size can currently be powered under the skin using a variety of methods. In deeper regions of the body, however, the device dimensions and robustness of powering are limited by the performance of the energy transfer system. Radiative and acoustic methods have been demonstrated for powering microdevices at a centimeter and beyond, but no transfer method yet can reliably power microdevices in arbitrary physiological regions. For very short durations of operation (a few minutes), such as for ingestible devices, batteries and galvanic cells remain viable powering options for microdevices.
Energy storage
The dominant technologies for implantable batteries are currently based on lithium chemistry (Li or Li ion) due to its high voltage and specific energy [40]. Conventional bulk batteries are challenging to miniaturize because they consist of composite electrodes and liquid electrolytes unsuited for microfabrication processes. Miniaturized battery (microbattery) technologies are based on solid-state electrolytes with thicknesses reduced to a few microns using thin-film or 3D architectures in order to overcome low ion conductivities. Commercial microbatteries can currently partially approach microscales (for example, 1.75 × 2.15 × 0.02 mm3, EnerChip, Cymbet Corporation) and provide performance sufficient for several applications, including an implantable orthodontic system [41]. However, the energy densities are very low (∼10 μAh), typically allowing only a few hours of active operation. The ability to recharge the battery through energy transfer [42] or harvesting [43,44] systems is needed to prolong their operational lifetimes. Although batteries are currently insufficient to power microdevices, they may play an important role in storing energy for short durations in order to address intermittency issues associated with energy harvesting or transfer technologies.
Energy transfer
Energy transfer methods are based on the transmission of electromagnetic or mechanical energy across intact biological tissue. The amount of power that can be transferred is constrained by the physical properties of tissue, which may result in heating, nonspecific stimulation or other adverse effects above thresholds for safety. Light can directly deliver energy into human body with versatile tunability and transduced into electrical power through the photovoltaic effect. Acoustic methods generally operate at ultrasound frequencies, owing to its safety, ability to be focused and clinical prevalence for imaging. Electromagnetic methods for energy transfer range from quasistatic to microwave frequencies, which interact with tissue and the device through distinct mechanisms. Although there are significant challenges that remain in efficiency, robustness and range, these energy transfer methods have been successfully used to power and operate microdevices in multiple configurations (Table 1).
Method | Limiting effect | Receiver type | Example microdevice |
---|---|---|---|
Electric | Tissue stimulation (<30 kHz), heating (>1 MHz) | Electrode | – |
Magnetic | Induced electric field | Coil | Brain implant ex vivo with 1 mm diameter coil and 2 cm intermediate resonator at 1.5 cm depth [73] |
Radiative | Tissue heating | Antenna | Cardiac pacing in vivo with 2 mm diameter coil at 5 cm depth [36] |
Optical | Tissue heating | Photovoltaic | Retinal implant in vivo with 70 μm pixels through transparent eye tissue [45] |
Acoustic | Tissue heating, mechanical effects (cavitation, acoustic streaming) | Piezoelectric | Neural recording invivo with 1 mm3 piezoelectric at 3 cm depth [46] |
Optical transfer
Light delivered into tissue can be converted to electrical power through the photovoltaic effect (Figure 2A) [47,48]. Lasers or light-emitting diodes can be used for illuminating tissue and integrated into potentially wearable devices. Light propagation through tissue is dominated by scattering and absorption, which limit the penetration depth. Visible light, for example, has a maximum penetration depth of 5 mm [49]. Wavelengths in the near-infrared biological ‘window’ (800–1000 nm) offer about an order of magnitude lower absorption, although the penetration depth remains limited to about a centimeter due to scattering [50]. Photovoltaic-based methods have been used to power retinal prostheses [51] and neural devices [52] with power densities of about 10 mW/cm2 at a few millimeters depth. Maintaining power levels below thresholds in order to minimize tissue heating is essential: general safety guidelines limit the thermal increase from 1°C in the eye [45] to 2°C on the skin [53]. Optical energy transfer approaches can currently power bioelectronic microdevices at the scale of a few hundred microns, but are limited to devices at a few millimeters depth in the body or in optically transparent regions such as the eye.

(A) A single photodiode of near-infrared-powered retinal prosthesis [48]. (B) Ultrasonically powered microdevice on the sciatic nerve in an anesthetized rat. Inset shows optional testing leads [17]. (C) Location-broadcasting device in which the frequency of the transmitted radiofrequency field depends on the magnetic field at the position of the device in analogy to MRI [54]. The device is compatible with wireless powering. (D) Passive pressure sensors for health monitoring which can be wirelessly interrogated at short range by inductive coils [55]. (E) Microdevice wirelessly powered by midfield techniques for cardiac pacing in pig models [56]. (i) Transmitter consisting of a phased surface in a curved state. (ii) Microdevice before encapsulation on human index finger. (iii) Computed tomography reconstruction of the transmitter powering the microdevice implanted in porcine upper abdomen.
Acoustic transfer
Acoustic waves at ultrasound frequencies (>20 kHz) have been widely investigated for energy transfer owing to its safety and low attenuation through tissue. The short wavelength of ultrasound enables focusing to millimeter-size regions in order to enhance efficiency and microscale resonant transducers for generating and receiving sound. The power levels are limited by thresholds for cavitation and thermal effects [57]. Scattering from hard–soft tissue interfaces, efficient coupling of transducers to tissue (typically mediated by gel), and the alignment of the device are major challenges, although many compensation methods have been developed [58,59]. The conversion of mechanical energy to electrical energy usually uses transducers based on bulk piezoelectrics, which can be small (<1 mm3) and provide high efficiencies of 70–90% (Figure 2B) [17,60]. Smaller transducers can be fabricated using piezoelectric micromachined ultrasound transducer technology, in which piezoelectric thin films are deposited on microscale diaphragms [61,62]. Ultrasound energy transfer has been clinically used to power cardiac devices (2.5 cm diameter, 9 mm length) using an implantable transmitter [63]. At the microscale, neural stimulators [64] and implantable oxygen generators [65] powered by ultrasound have been demonstrated in rodents. Although ultrasound can routinely access regions of the body at many centimeters of depth for imaging, its use in powering microdevices at human scales has not yet been demonstrated and will require challenges in scattering, orientation and coupling at the tissue interface to be overcome.
Magnetoquasistatic transfer
Much attention has been devoted to the use of magnetic fields for energy transfer owing to the nonmagnetic properties of biological tissue [54,66] (Figure 2C). Pairs of coils coupled through magnetic induction have been used for wireless powering of implantable medical devices since the early 1960s [67], and are widely used in clinical devices such as cochlear implants today [2]. All methods are based on magnetic induction with a time-varying magnetic field (1 kHz to 100 MHz) and involve a nonzero electric field throughout tissue [68], which limits the maximum amount of power that can be safely transmitted. At frequencies below 30 kHz where stimulatory effects dominate, general safety guidelines constrain the electric field amplitude (<2 V/m), while at higher frequencies where heating losses dominate, the specific absorption rate is limited (<2 W/kg) [69]. Limits for clinical systems require weighing of the risks and benefits as the guidelines are not applicable to medical use.
The transfer efficiency can be significantly enhanced by resonating the coils at the same frequency and tuning the rates of energy dissipation (impedance matching) [70–72]. The coupled system, however, exhibits a frequency response different from the individual coils (frequency splitting), and results in a coupling-dependent efficiency that is sensitive to the coil placement and natural physiological motion. Significant effort has been devoted to designing compensation circuits, which mitigate this variation in order to provide robust power transfer in dynamic environments [73,74].
The miniaturization of the device is primarily limited by weak coupling to the source coil. The coupling depends on the degree of overlap between the generated field and device, and consequently decreases with the size of the device and the distance from the source [75]. The field amplitude is subject to reactive field decay with distance from the source, independently from the properties of tissue [76]. These coupling considerations typically limit the size of the device to dimensions comparable to the depth of implantation. The degree to which resonant enhancement can compensate for weak coupling is limited by losses in the system, which at low frequencies are typically dominated by resistive losses in the coils [77]. At present, microdevices wirelessly powered by magnetoquasistatic fields are feasible for implantation at a few millimeters depth, but low efficiencies hinder its use for deeper implants. The use of an intermediate device to relay energy provides a potential route to powering deeper microdevices, but currently requires implantation of a centimeter-scale coil.
Radiative transfer
Radiative approaches use electromagnetic waves for energy transfer. The wave-like nature of electromagnetic fields becomes significant when the wavelength is smaller than the scale of the human body, generally when the operating frequency exceeds about 500 MHz. Both the electric and magnetic components of the radiative field are significant with ratio set by the medium of propagation, independent of the design of the source. Owing to radiative energy transport, the field decay is dominated by tissue absorption rather than reactive decay. Because of the high frequency of operation, the primary interaction with tissue is heating from the electric field component of the wave [78].
Devices implanted near the surface of the body can be powered by radiofrequency waves at a distance. Using a conventional antenna, an intraocular device (about 1 cm diameter) was powered at a distance of 10 cm for continuous pressure monitoring [79]. Powering deep devices typically requires placement of the source close to the body surface to avoid strong reflection at the air–tissue boundary. Because of the high dielectric permittivity of biological tissues, the wavelength is significantly shorter in tissue than in free space (by a factor of 2–7, depending on the type of tissue). The receiver can be designed for interaction with the electric or magnetic field component, or both (Figure 2D) [55].
The transfer efficiency can be enhanced by reshaping the field distribution through interference. In analogy to far-field wireless systems, antenna design techniques can be used to tune the directionality of radiation preferentially in the direction of the microdevice. Midfield techniques exploit the short distance between the transmitter and the device (few wavelengths) in order to shape the 3D field distribution. Optimization of the phase of currents on the surface of the body can focus the field several centimeters deep to power miniaturized devices [74,80]. Wireless powering of microstimulators (about 2 mm in size) was demonstrated for cardiac pacing in large animals (about 5 cm deep) using average transmit power levels of 200 mW (compared with 500 mW for typical cellular phones) (Figure 2E) [36,56], representing the demonstration of an operational microdevice at a substantially greater depth than achieved with other electromagnetic approaches. However, theoretical studies suggest that this performance approaches theoretical limits for the degree of enhancement that can be achieved from reshaping the field distribution [81].
Galvanic cells
Galvanic cells harness electrochemical reactions from the dissolution of electrodes in physiological fluid. Much work has focused on powering ingestible devices in which a dissolving metal anode undergoes oxidation, a cathode returns electrons to the solution and gastric fluid acts as the electrolyte. The most widely studied anode materials are Mg and Zn owing to their manufacturability and dietary role. The lifetime of the device depends on the amount of electrode material. Zn electrodes with area of about 10 mm2 generated 0.1–1 μW/mm2 of power in pig stomach over the duration of a week, sufficient to power pulsatile transmission of wireless signals from a centimeter-sized capsule (Figure 4A) [80]. Microdevices capable of generating measurable surface electric potentials for communication can be powered for a few minutes using Mg-Cu cells with an area of 1 mm2 [82]. Pharmaceutical tablets integrated with these microdevices are already commercially available for measuring medical adherence (Proteus Digital Health).
Emerging powering methods
A diverse range of other methods have been investigated for use in powering biomedical devices. In this section, we briefly overview emerging powering methods that are relevant for microdevices. Although current technologies based on these approaches do not yet meet requirements for autonomously powering a microdevice, they may complement the energy source for existing microdevices and, with continued advances, may emerge as viable powering options.
Energy harvesting from physiological processes of human body
Physiological processes such as metabolism, respiration, locomotion and circulation generate diverse forms of energy locally and globally in the human body (Figure 3). Energy harvesting methods convert such mechanical, thermal, bioelectrical and biochemical energy sources into electrical power. Although significant advances have been made over the last decade, these energy methods generally achieve low power densities <0.1 μW/mm2 and still require further improvement in order to meet the basic requirements of microdevices (Table 2). Nevertheless, the ability of energy-harvesting methods to generate power in absence of an external source can complement current energy transfer and storage approaches in order to prolong the lifetimes and enhance the reliability of microdevice powering.

Schematic of energy harvesting based on (A) galvanic cell, (B) piezoelectric methods, (C) triboelectric methods and (D) thermoelectric methods, (E) biopotential cell and (F) biofuel cell.
Type | Area (mm2) | Thickness (mm) | In vivo performance | Energy harvested | ||
---|---|---|---|---|---|---|
Voltage (V) | Current (μA) | Power density (mW/m2) | ||||
Triboelectric | 144–600 | 1–1.5 | 3.73–14 | 0.14–5 | 8.44–107 | Heartbeat [83,84], breathing [85] |
Piezoelectric | 100–1120 | 0.1–0.2 | 1.5–3.7 | 0.06–0.3 | 0.1–1.2 | Blood flow [86], cardiac motion [44,87] |
Biofuel cell | 2–133 | 10 | 0.192–0.275 | 0.03–10 | 24.4–1800 | Physiological fluid [88], cerebrospinal fluid [89] |
Galvanic cell | 78.5 | 30 | 0.23 | – | 230 | Oxidation of Zn [80] |
Biopotential | 99 | 0.2 | 0.03–0.055 | 0.8–28 | 1.1–6.3 nW | Endocochlear potential [90] |
Thermoelectric | 67.9–225 | 1.4–9 | 3.84–520 mV/K | ∼2 μA/K | ∼6 μW/K | Heat transfer on rabbit's cecum [91], generic [92] |
Piezoelectrics
Piezoelectric materials perform reversible mechanical-to-electrical energy conversion through the linear interaction between their electrical and mechanical crystal states (Figure 4B) [87,93]. Materials for energy harvesting include the lead zirconate titanate and lead-free alternatives such as BaTiO3, Na0.5Bi0.5TiO3, and K0.5Bi0.5TiO3 for their nontoxicity and high piezoelectric coefficient [94]. Minimizing the mechanical mismatch between the harvesting device and soft moving tissues is critical for performance and safety. Building on early work with piezoelectric beams [95], flexible piezoelectric harvesting devices have been demonstrated using flexible substrates layered with piezoelectric nanowires or thin films. Proof-of-concept devices deployed on the surface of the heart in large animals yield 1.2 μW/cm2, sufficient to pace the heart with a centimeter-scale flexible implant [44], although long-term evaluation is still needed.

(A) Ingestible device (10 mm diameter × 30 mm length) powered by a galvanic cell in porcine stomach [80]. (B) Piezoelectric energy harvesting of swine gastrointestinal motion. The device dimensions are 25 mm × 20 mm × 0.08 mm [93]. (C) Triboelectric energy harvesting of respiratory in rat diaphragm with a 12 mm × 12 mm device [85]. (D) Thermoelectric energy harvesting scheme [91]. (E) Microdevice powered by endocochlear potential [90]. (F) Harvesting energy from rat body fluid by biofuel cell [96].
Triboelectrics
Frictional transfer of surface charge during contact between materials of opposite triboelectric polarity provides another mechanism for mechanical energy harvesting [97]. The conversion efficiency of triboelectric harvesters depends on the surface work functions of the materials and the structure of the contact surface. Devices based on contact areas of >5 cm2 between nanostructured elastomer surfaces and Au film have been demonstrated for powering devices using energy from respiration (Figure 4C) [85], cardiac activity [83] and motion [98]. Most recently, flexible triboelectric devices have been successfully demonstrated for an electronic auditory system for potential use in aiding hearing-impaired patients [99], although the dimensions are also limited to several centimeters.
Thermoelectrics
Thermal gradients generated by normal metabolism have been exploited for powering devices. Conversion relies on the thermoelectric effect and the performance of the devices depends on the temperature gradient, materials properties and the configuration of modules (Figure 4D) [91,100]. Most work has focused on wearable devices in order to harness the large thermal gradient between the body and the surrounding environment, which can generate approximately 100 μW/cm2 under indoor conditions [101]. The low thermal gradient of the physiological environment at the microscale currently limits the use of thermoelectrics for miniaturized devices.
Biopotentials
Electrochemical potentials maintained by the body can also power devices in specific anatomical regions. The largest direct current potential in mammals is the endocochlear potential (70–100 mV) maintained by the inner ear. Using a 1 cm2 device, extraction of 1.12 nW has been demonstrated in animals, sufficient to transmit a wireless signal with an interval of few minutes (Figure 4E) [90]. Biopotentials are maintained across many other organs, including the heart, brain and retina, as well as at the cellular level across cell membranes, and remain largely unexplored for powering microdevices.
Fuel cells
Biofuel cells are an important type of fuel cell that generates electricity from the catalytic reaction of biochemical fuels, such as glucose, that are readily available in the human body. The chemical energy of glucose can be harvested to power bioelectronic devices via the catalytic oxidation of glucose at an anode and reduction of oxygen at a cathode. Biofuel cells can be further categorized by the choice of biocatalyst into enzymatic [102] or microbial [103,104] types, which has been extensively investigated for applications to biomedical devices.
Fuel cells may also utilize abiotic catalytic materials in order to circumvent degradation and biocompatibility concerns associated with biocatalysts [105,106]. Such pure inorganic fuel cells typically achieve lower efficiencies (40 μW/cm2) [107] compared with enzymatic biofuel cells (193.5 μW/cm2) [96] in in vivo demonstrations (Figure 4F), but feature longer lifetimes and improved biocompatibility [108]. However, integration with inorganic structures, such as nanotubes, nanoparticles and nanofibers, can largely advance the power density of enzymatic biofuel cells due to their large surface areas, high aspect ratios and high conductivities [109]. In addition, the integration of these nanostructures with enzyme can enhance the rate of electron transfer between an enzyme and an electrode after immobilization of mediators on them.
Fuel cells have been demonstrated for powering implanted devices as well as wearable devices [110]. The availability of glucose and oxygen as well as the biofouling is an important issue for implantable device performance [111]; studies have focused on blood [105], interstitial fluids [88] and cerebrospinal fluid [89]. Proof-of-concept animal studies have been performed using centimeter-scale devices, although there is still space for further miniaturization and improvement of long-term performance.
Other energy transfer methods
Capacitive (electroquasistatic) transfer
The strong interaction of electric fields with tissue, arising from the polarizability and ionic conductivity of biological matter, poses challenges for its use in energy transfer. Methods are nonetheless feasible if the amplitude of the field is subject to stringent limitations (<2 V/m) to avoid nonspecific stimulation of electrically excitable tissues [69]. The most prominent example is capacitive coupling, which utilizes a time-varying (1 kHz to 1 MHz) electric field to induce an electromotive force in an implanted electrode that is proportional to both the field strength and the length of the electrode. The field amplitude from the external electrode is subject to reactive field decay and limits the miniaturization of the device to dimensions comparable to the transfer depth [112,113]. Other effects that must be considered for safety include heating of tissue by dielectric losses and voltage thresholds for redox reactions on electrode surfaces (faradaic currents). In demonstrations so far, the size of the implanted electrode has been limited to a few centimeters in order to achieve subcutaneous powering.
Hybrid methods
Hybrid methods combine electromagnetic and mechanical interactions for power transfer. An example is the magnetoacoustic power transfer in which a time-varying magnetic field is transduced into mechanical vibrations by the magnetostriction of ferromagnetic materials, which is subsequently converted to electrical power by piezoelectrics. Proof-of-concept experiments have been demonstrated [114,115], although integration with an implantable microdevice has yet to be performed.
Energy storage with supercapacitors
Supercapacitors are an emerging energy storage technology, which exhibit superior power densities and can complement energy transfer or harvesting technologies by storing excess power. In contrast to batteries (Figure 5), supercapacitors store energy either electrostatically through ion adsorption (electrochemical double-layer capacitor) or through rapid surface redox reactions (pseudocapacitor). They are being intensively studied as alternatives to batteries for energy storage due to their safety, rapid charging/discharging times, and nearly unlimited number of charging cycles [116] (Table 3). Although the energy density of supercapacitors is lower than that of batteries, these properties provide distinct advantages for storing energy intermittently obtained by harvesting [117,118] or transfer systems [119–122].

Batteries store energy electrochemically and supercapacitors electrostatically (electrochemical double-layer capacitor) or through fast surface redox reactions (pseudocapacitor) [123].
Type | Area (mm2) | Thickness (mm) | Rated capacity (mAh) | Discharge current (mA) | Energy (μWh/cm2) | Power (mW/cm2) | Cycles | Ref. |
---|---|---|---|---|---|---|---|---|
Primary microbatteries | 18–78 | 1.2–2.5 | 1–30 | 0.1–0.8 | – | – | 1 | [124] |
Thin-film batteries | 2–1000 | 0.00005–0.02 | 0.005–0.9 | 0.003–10 | 0.0425–1700 | 0.7–50 | 15–40,000 | [41,124,125] |
2D microsupercapacitor | – | – | – | – | 0.07–50 | 30–1300 | 10,000–105,000 | [126–128] |
3D microsupercapacitor | – | – | – | – | 49–126 | 7.9–34.4 | ∼2000 | [129–131] |
The miniaturization of supercapacitors using silicon fabrication techniques has enabled devices with microscale form factors [132]. Such ‘microsupercapacitors’ have been developed in planar configurations using interdigitated electrode geometries with areas from 0.1 to 10 cm2 and volumes from 0.01 to 1 cm3 [133,134], and can be thin and flexible [135]. To prevent leakage of the microsupercapacitor, solid-state or gel-type electrolytes, especially ionogels are preferred [136,137]. About tenfold higher energy densities can be achieved with 3D electrode architectures in which the anodes and cathodes are exposed in three dimensions, although at the expense of lower power densities (on the order of 10 mW/cm2 compared with 100–1000 mW/cm2) [132]. Compact integration of microsupercapacitors with bioelectronic devices with dimensions <1 mm2 has been demonstrated using architectures compatible with surface mounting (Figure 6) [138].

(A) Photograph of microbattery integrated in a piezoelectric system that was implanted on a bovine's lung [44]. (B) Photographs of ultrasmall integrated 3D microsupercapacitor (i) topside; (ii) backside; (iii) inset of single device (top side); (iv) inset of single device (backside) [138].
Conclusion & future perspective
Despite the wide variety of powering methods being developed, bioelectronic microdevices can currently be powered only under limited circumstances. Short duration of active operation is possible with batteries, supercapacitors and, in the specific environment of the stomach, galvanic cells. Longer term operation of microdevices can currently be achieved in certain configurations using energy transfer approaches. At a few millimeters below the skin, near-infrared light and magnetic induction can transfer useful levels of power to microdevices. The range can be safely extended to about 5 cm deep using radiative approaches that focus the electromagnetic field within the body. Ultrasound energy transfer promises the ability to deliver higher power densities and proof-of-concept has been demonstrated in small animals, but advancing to human-scale demonstrations will require challenges in scattering and reliability to be overcome. The development of systems that can adaptively reshape the field distribution inside the body in response to physiological changes and also be conveniently worn on the body represents an important research direction for improving energy transfer performance and robustness.
Although significant advances are being made in energy-harvesting methods, the power densities achieved so far are too low to power a microdevice. Further progress in low-power bioelectronics may also enable power requirements to be relaxed: a key challenge is communication with external devices, which remains power-intensive relative to other functions. Proof-of-concept microdevices demonstrated so far are all at the scale of a single millimeter. Miniaturization to the scale of 100 μm, which enables needle injection and evasion of the immune response, remains a major goal. Combining energy transfer approaches with harvesting and storage technologies provides a promising direction for reliable powering of microdevices throughout the human body.
Owing to their relative ease of implantation, applications of microdevices may depart from traditional uses of medical devices. Microdevices are likely to first be used in applications where they can be powered on-demand rather than continuously, such as for transient stimulation or sensor readout. Such applications are well suited for powering using energy transfer methods since the external source can be removed after use. Active interaction between the patient and the device, such as with today's wirelessly programmable stimulators, may be a common mode of usage. Multiple devices could also be implanted such that failure of a single device does not compromise efficacy. New materials may also enable microdevices to function temporarily in the body and be resorbed without need for retrieval [139]. The development of advanced powering platforms for microdevices should anticipate these new modes of usage.
The ultimate goal of bioelectronic microdevices is to develop minimally invasive interfaces for monitoring and modulating physiological activity with microscale spatial precision and continuous temporal resolution. These capabilities are a key to realizing major goals in biomedicine, from brain mapping to personalized medicine. Although fundamental challenges remain in the powering of these devices, continued progress in the transfer, harvesting and storage of energy within the body as well as systems that exploit the synergies between them will accelerate the development of microdevices and enable new approaches to diagnose and treat disease.
Executive summary
This review describes methods for powering bioelectronic microdevices – devices capable of functioning autonomously at the scale of a millimeter or less – through approaches that involve the storage, transfer or harvesting energy in the environment of the human body.
The scaling of electronics has enabled microdevices to be endowed with sophisticated sensing, actuation and signal processing capabilities, but powering these devices in the physiological environment remains a major technological challenge.
Microdevices powered by batteries or galvanic cells can operate in the body for short durations (minutes to hours), although limitations in energy storage technology constrain their long-term use.
Currently, methods to transfer energy through magnetic, radiative, optical or acoustic means have enabled the demonstration of microdevices in various physiological environments for applications in stimulation and monitoring. Continued advances, however, are needed to reliably power microdevices in all regions of the body.
Emerging approaches to power microdevices include strategies to harvest mechanical, thermal, bioelectrical and biochemical energy in the human body. These do not yet meet requirements for continuously powering a microdevice, but can complement energy transfer and storage methods.
Microdevices promise new capabilities in minimally invasive monitoring and modulation of physiological activity for medicine. Advances in combined energy storage, transfer and harvesting approaches will enable such devices to be powered.
Financial & competing interests disclosure
The authors have no relevant affiliations or financial involvement with any organization or entity with a financial interest in or financial conflict with the subject matter or materials discussed in the manuscript apart from those disclosed.
No writing assistance was utilized in the production of this manuscript.
Open access
This work is licensed under the Attribution-NonCommercial-NoDerivatives 4.0 Unported License. To view a copy of this license, visit http://creativecommons.org/licenses/by-nc-nd/4.0
Papers of special note have been highlighted as: • of interest
References
- 1 . A surgical approach to the management of heart-block using an inductive coupled artificial cardiac pacemaker. Lancet 275(7139), 1372–1374 (1960).
- 2 . Cochlear implants: system design, integration, and evaluation. IEEE Rev. Biomed. Eng. 1, 115–142 (2008).
- 3 An ingestible bacterial-electronic system to monitor gastrointestinal health. Science 360(6391), 915–918 (2018).
- 4 . Wireless capsule endoscopy: from diagnostic devices to multipurpose robotic systems. Biomed. Microdevices 9(2), 235–243 (2007).
- 5 . Mesh nanoelectronics: seamless integration of electronics with tissues. Acc. Chem. Res. 51(2), 309–318 (2018).
- 6 . Neuroprosthetics: restoring multi-joint motor control. Nat. Biomed. Eng. 1, 0073 (2017).
- 7 . The effect of microgeometry, implant thickness and polyurethane chemistry on the foreign body response to subcutaneous implants. Biomaterials 23(21), 4185–4192 (2002).
- 8 . The NIH brain initiative. Science 340(6133), 687–688 (2013).
- 9 Bioelectronic medicines: a research roadmap. Nat. Rev. Drug Discov. 13(6), 399 (2014).
- 10 Preparation and implementation of optofluidic neural probes for in vivo wireless pharmacology and optogenetics. Nat. Protocols 12, 219 (2017).
- 11 . Light-driven micro/nanomotor for promising biomedical tools: principle, challenge, and prospect. Acc. Chem. Res.
doi:10.1021/acs.accounts.8b00254 (2018) (Epub ahead of print). - 12 A silicon nanowire as a spectrally tunable light-driven nanomotor. Adv. Mater. 29(30), 1701451 (2017).
- 13 Rational design of silicon structures for optically controlled multiscale biointerfaces. Nat. Biomed. Eng. 2(7), 508–521 (2018).
- 14 Microscale optoelectronic infrared-to-visible upconversion devices and their use as injectable light sources. Proc. Natl Acad. Sci. USA
doi:10.1073/pnas.1802064115 (2018) (Epub ahead of print). • Demonstrates infrared energy transfer to microdevices for optical brain stimulation. - 15 A microscale optical implant for continuous in vivo monitoring of intraocular pressure. Microsystems Nanoengineering 3, 17057 (2017).
- 16 . Ultralow-power electronics for biomedical applications. Ann. Rev. Biomed. Eng. 10(1), 247–274 (2008). • Summarizes the power requirements of biomedical electronic devices.
- 17 Wireless recording in the peripheral nervous system with ultrasonic neural dust. Neuron 91(3), 529–539 (2016).
- 18 . Neurons to silicon: implantable prosthesis processor. Presented at: Solid-State Circuits Conference, 2006. ISSCC 2006. Digest of Technical Papers. IEEE International. San Francisco, CA, USA, 6–9 February (2006).
- 19 . A fully-integrated 10.5μw miniaturized (0.125mm2) wireless neural sensor. Presented at: 2012 Symposium on VLSI Circuits (VLSIC). Hilton Hawaiian Village, Honolulu, HI, USA, 12–15 June (2012).
- 20 . Characterization of two-turns external loop antenna with magnetic core for efficient wireless powering of cortical implants. IEEE Antennas Wireless Propag. Lett. 15, 1410–1413 (2016).
- 21 . On-chip implantable antennas for wireless power and data transfer in a glaucoma-monitoring SoC. IEEE Antennas Wireless Propag. Lett. 11, 1671–1674 (2012).
- 22 . A 0.013 mm, 5 uW, DC-coupled neural signal acquisition IC with 0.5 V supply. IEEE J. Solid-State Circuits 47(1), 2167–2176 (2016).
- 23 . 256-site active neural probe and 64-channel responsive cortical stimulator. Presented at: Custom Integrated Circuits Conference (CICC), 2011 IEEE. San Jose, CA, USA, 19–21 September (2011).
- 24 A 128-channel 6mW wireless neural recording IC with on-the-fly spike sorting and UWB tansmitter. Presented at: Solid-State Circuits Conference, 2008. ISSCC 2008. Digest of Technical Papers. IEEE International. San Francisco, CA, USA, 3–7 February (2008).
- 25 . Electrical impedance behavior of biological tissues during transcutaneous electrical stimulation. Braz. J. Phys. Ther. 11(2), 153–159 (2007).
- 26 . Variation in deep brain stimulation electrode impedance over years following electrode implantation. Stereotact. Funct. Neurosurg. 92(2), 94–102 (2014).
- 27 . Constant-current deep brain stimulation improves symptoms in Parkinson disease. Nat. Rev. Neurol. 8, 119 (2012).
- 28 . The 128-channel fully differential digital integrated neural recording and stimulation interface. IEEE Trans. Biomed. Circuits Syst. 4(3), 149–161 (2010).
- 29 . A power-efficient neural tissue stimulator with energy recovery. IEEE Trans. Biomed. Circuits Syst. 5(1), 20–29 (2011).
- 30 . A flexible, portable system for neuromuscular stimulation in the paralyzed upper extremity. IEEE Trans. Biomed. Eng. 35(11), 897–904 (1988).
- 31 . Wireless power transfer by inductive coupling for implantable batteryless stimulators. Presented at: Microwave Symposium Digest (MTT), 2012 IEEE MTT-S International. Montreal, QC, Canada, 17–22 June (2012).
- 32 . A very low-power CMOS mixed-signal IC for implantable pacemaker applications. IEEE J. Solid-State Circuits 39(12), 2446–2456 (2004).
- 33 Inductively powered wireless pacing via a miniature pacemaker and remote stimulation control system. Sci. Rep. 7(1), 6180 (2017).
- 34 A complete 256-electrode retinal prosthesis chip. IEEE J. Solid-State Circuits 49(3), 751–765 (2014).
- 35 A fully intraocular high-density self-calibrating epiretinal prosthesis. IEEE Trans. Biomed. Circuits Syst. 7(6), 747–760 (2013).
- 36 Wireless power transfer to deep-tissue microimplants. Proc. Natl Acad. Sci. USA 111(22), 7974–7979 (2014).
- 37 . In vivo wireless photonic photodynamic therapy. Proc. Natl Acad. Sci. USA
doi:10.1073/pnas.1717552115 (2018) (Epub ahead of print). - 38 . Electrothermal microactuators with peg drive improve performance for brain implant applications. J. Microelectromech. Syst. 21(5), 1172–1186 (2012).
- 39 A novel micro-fabricated thruster for drug release in remote controlled capsule. Sens. Actuators A 159(2), 227–232 (2010).
- 40 . Batteries used to power implantable biomedical devices. Electrochimica Acta 84, 155–164 (2012).
- 41 Flexible and biocompatible high-performance solid-state micro-battery for implantable orthodontic system. npj Flexible Electronics 1(1), 7 (2017).
- 42 . A wireless power interface for rechargeable battery operated neural recording implants. Presented at: Engineering in Medicine and Biology Society, 2006. EMBS'06. 28th Annual International Conference of the IEEE. NY, USA (30 August–3 September 2006).
- 43 . Piezoelectric rubber films for human physiological monitoring and energy harvesting. Presented at: 2013 IEEE 26th International Conference on Micro Electro Mechanical Systems (MEMS). Taipei, Taiwan, January (2013).
- 44 Conformal piezoelectric energy harvesting and storage from motions of the heart, lung, and diaphragm. Proc. Natl Acad. Sci. USA 111(5), 1927–1932 (2014).
- 45 Photovoltaic retinal prosthesis with high pixel density. Nat. Photonics 6(6), 391 (2012).
- 46 . A mm-sized implantable medical device (IMD) with ultrasonic power transfer and a hybrid bi-directional data link. IEEE J. Solid-State Circuits 50(8), 1741–1753 (2015).
- 47 Photoelectrochemical modulation of neuronal activity with free-standing coaxial silicon nanowires. Nat. Nanotechnol. 13(3), 260–266 (2018).
- 48 Photovoltaic restoration of sight with high visual acuity. Nat. Med. 21, 476 (2015).
- 49 . Measurements of light penetration into human tissues in vivo. Presented at: Photochemotherapy: Photodynamic Therapy and Other Modalities. BiOS Europe ‘95, 1995, Barcelona, Spain (1996).
- 50 . Effect of wavelength and beam width on penetration in light-tissue interaction using computational methods. Lasers Med. Sci. 32(8), 1909–1918 (2017).
- 51 Performance of photovoltaic arrays in vivo and characteristics of prosthetic vision in animals with retinal degeneration. Vision Res. 111, 142–148 (2015).
- 52 1 mm3-sized optical neural stimulator based on CMOS integrated photovoltaic power receiver. AIP Advances 8(4), 045018 (2018).
- 53 . An implantable power supply with an optically rechargeable lithium battery. IEEE Trans. Biomed. Eng. 48(7), 830–833 (2001).
- 54 . Localization of microscale devices in vivo using addressable transmitters operated as magnetic spins. Nat. Biomed. Eng. 1(9), 736 (2017).
- 55 Continuous wireless pressure monitoring and mapping with ultra-small passive sensors for health monitoring and critical care. Nat. Commun. 5, 5028 (2014). • Demonstrates inductively coupled microdevices for pressure sensing.
- 56 Conformal phased surfaces for wireless powering of bioelectronic microdevices. Nat. Biomed. Eng. 1(3), 0043 (2017). • Demonstrates midfield energy transfer to deep microdevices for cardiac stimulation.
- 57 . The sensitivity of biological tissue to ultrasound. Ultrasound Med. Biol. 23(6), 805–812 (1997).
- 58 A flexible ultrasound transducer array with micro-machined bulk PZT. Sensors 15(2), 2538–2547 (2015).
- 59 . Compensating for tissue changes in an ultrasonic power link for implanted medical devices. IEEE Trans. Biomed. Circuits Syst. 10(2), 404–411 (2016).
- 60 . Efficiency of energy conversion for devices containing a piezoelectric component. J. Micromechanics Microengineering 14(5), 717 (2004).
- 61 . Ultrasonically powered piezoelectric generators for bio-implantable sensors: plate versus diaphragm. J. Intelligent Mater. Syst. Structures 27(8), 1092–1105 (2016).
- 62 . Through-package wireless powering via piezoelectric micromachined ultrasonic transducers. Presented at: 2018 IEEE Micro Electro Mechanical Systems (MEMS). Belfast, UK, 21–25 January (2018).
- 63 First-in-man implantation of leadless ultrasound-based cardiac stimulation pacing system: novel endocardial left ventricular resynchronization therapy in heart failure patients. Europace 15(8), 1191–1197 (2013).
- 64 . An ultrasonically-driven piezoelectric neural stimulator. Presented at: Engineering in Medicine and Biology Society, 2003. Proceedings of the 25th Annual International Conference of the IEEE. Cancun, Mexico, 17–21 September (2003).
- 65 . An ultrasonically powered implantable micro-oxygen generator (IMOG). IEEE Trans. Biomed. Eng. 58(11), 3104–3111 (2011).
- 66 . Midfield wireless powering for implantable systems. Proc. IEEE 101(6), 1369–1378 (2013).
- 67 . Powering an artificial heart: birth of the inductively coupled-radio frequency system in 1960. Artif. Organs 26(11), 909–915 (2002).
- 68 . Mutual inductance of air-cored coils: effect on design of radio-frequency coupled implants. Med. Biol. Eng. 9(2), 79–85 (1971).
- 69 IEEE standard for safety levels with respect to human exposure to radio frequency electromagnetic fields, 3 kHz to 300 GHz. IEEE Std C95.1–2005 (Revision of IEEE Std C95.1–1991)
doi:10.1109/IEEESTD.2006.99501 (2006) (Epub ahead of print). - 70 . Design and optimization of printed spiral coils for efficient transcutaneous inductive power transmission. IIEEE Trans. Biomed. Circuits Syst. 1(3), 193–202 (2007).
- 71 . Powering a ventricular assist device (VAD) with the free-range resonant electrical energy delivery (FREE-D) system. Proc. IEEE 100(1), 138–149 (2012).
- 72 . Wireless power transmission with self-regulated output voltage for biomedical implant. IEEE Trans. Ind. Electronics 61(5), 2225–2235 (2014).
- 73 . Optimal design of wireless power transmission links for millimeter-sized biomedical implants. IEEE Trans. Biomed. Circuits Syst. 10(1), 125–137 (2016). • Demonstrates magnetoquasistatic energy transfer to brain microdevices.
- 74 . Robust wireless power transmission to mm-sized free-floating distributed implants. IEEE Trans. Biomed. Circuits Syst. 11(3), 692–702 (2017).
- 75 . Design of radio-frequency powered coils for implant instruments. Med. Biol. Eng. Comput. 15(6), 634–640 (1977).
- 76 . Design and optimization of resonance-based efficient wireless power delivery systems for biomedical implants. IEEE Trans. Biomed. Circuits Syst. 5(1), 48–63 (2011).
- 77 . Inductive power transfer. Proc. IEEE 101(6), 1276–1289 (2013).
- 78 . Far-field RF powering of implantable devices: safety considerations. IEEE Trans. Biomed. Eng. 60(8), 2107–2112 (2013).
- 79 . A miniature-implantable RF-wireless active glaucoma intraocular pressure monitor. IEEE Trans. Biomed. Circuits Syst. 4(6), 340–349 (2010).
- 80 Prolonged energy harvesting for ingestible devices. Nat. Biomed. Eng. 1(3), 0022 (2017).
- 81 . Midfield wireless powering of subwavelength autonomous devices. Phys. Rev. Lett. 110(20), 203905 (2013).
- 82 . An ingestible sensor for measuring medication adherence. IEEE Trans. Biomed. Eng. 62(1), 99–109 (2015). • Demonstrates powering of an ingestible microdevice with a galvanic cell for communication.
- 83 In vivo self-powered wireless cardiac monitoring via implantable triboelectric nanogenerator. ACS Nano 10(7), 6510–6518 (2016).
- 84 . In vitro and ex vivo strategies for intracellular delivery. Nature 538, 183 (2016).
- 85 In vivo powering of pacemaker by breathing-driven implanted triboelectric nanogenerator. Adv. Mater. 26(33), 5851–5856 (2014).
- 86 A flexible and implantable piezoelectric generator harvesting energy from the pulsation of ascending aorta: in vitro and in vivo studies. Nano Energy 12, 296–304 (2015).
- 87 Self-powered cardiac pacemaker enabled by flexible single crystalline PMN-PT piezoelectric energy harvester. Adv. Mater. 26(28), 4880–4887 (2014).
- 88 A glucose biofuel cell implanted in rats. PLoS ONE 5(5), e10476 (2010).
- 89 . A glucose fuel cell for implantable brain–machine interfaces. PLoS ONE 7(6), e38436 (2012).
- 90 . Energy extraction from the biologic battery in the inner ear. Nat. Biotechnol. 30(12), 1240 (2012).
- 91 . Suitability of a thermoelectric power generator for implantable medical electronic devices. J. Phys. D 40(18), 5790 (2007).
- 92 . Invited talk: thermal energy harvesting with thermo life. Presented at: International Workshop on Wearable and Implantable Body Sensor Networks, 2006. BSN 2006. Cambridge, MA, USA, 3–5 April (2006).
- 93 Flexible piezoelectric devices for gastrointestinal motility sensing. Nat. Biomed. Eng. 1(10), 807–817 (2017).
- 94 . Advances in lead-free piezoelectric materials for sensors and actuators. Sensors 10(3), 1935–1954 (2010).
- 95 . Powering pacemakers from heartbeat vibrations using linear and nonlinear energy harvesters. Appl. Phys. Lett. 100(4), 042901 (2012).
- 96 Single glucose biofuel cells implanted in rats power electronic devices. Sci. Rep. 3, 1516 (2013).
- 97 . Triboelectric nanogenerators as flexible power sources. npj Flexible Electronics 1(1), 10 (2017).
- 98 Implantable self-powered low-level laser cure system for mouse embryonic osteoblasts’ proliferation and differentiation. ACS Nano 9(8), 7867–7873 (2015).
- 99 A highly sensitive, self-powered triboelectric auditory sensor for social robotics and hearing aids. Sci. Robotics 3(20), 1–10 (2018).
- 100 . Kinetic and thermal energy harvesters for implantable medical devices and biomedical autonomous sensors. Meas. Sci. Technol. 25(1), 012003 (2013).
- 101 . Wearable thermoelectric generators for body-powered devices. J. Electronic Mater. 38(7), 1491–1498 (2009).
- 102 . Nanostructured material-based biofuel cells: recent advances and future prospects. Chem. Soc. Rev. 46(5), 1545–1564 (2017).
- 103 . Miniaturizing microbial fuel cells for potential portable power sources: promises and challenges. Microfluidics Nanofluidics 13(3), 353–381 (2012).
- 104 . Miniaturizing microbial fuel cells. Trends Biotechnol. 29(2), 62–69 (2011).
- 105 . Energy harvesting by implantable abiotically catalyzed glucose fuel cells. J. Power Sources 182(1), 1–17 (2008).
- 106 . The applications and prospect of fuel cells in medical field: a review. Renewable Sustainable Energy Rev. 67, 574–580 (2017).
- 107 . Implanted fuel-cell for operation of mechanical heart. Berichte der Bunsen-Gesellschaft – Phys. Chem. Chem. Phys. 77, 782–783 (1973).
- 108 . Towards glucose biofuel cells implanted in human body for powering artificial organs: review. Electrochemistry Commun. 38, 19–23 (2014).
- 109 . Nanostructured inorganic materials at work in electrochemical sensing and biofuel cells. Catalysts 7(1), 31 (2017).
- 110 . Enzymatic fuel cells: towards self-powered implantable and wearable diagnostics. Biosensors 8(1), 11 (2018).
- 111 . New materials for biological fuel cells. Mater. Today 15(4), 166–173 (2012).
- 112 . Wireless power delivery to flexible subcutaneous implants using capacitive coupling. IEEE Trans. Microwave Theory Tech. 65(1), 280–292 (2017).
- 113 . Electric near-field coupling for wireless power transfer in biomedical applications. Presented at: 2013 IEEE MTT-S International Microwave Workshop Series on RF and Wireless Technologies for Biomedical and Healthcare Applications (IMWS-BIO). Singapore, Singapore, 9–11 December (2013).
- 114 . Low frequency wireless powering of microsystems using piezoelectric–magnetostrictive laminate composites. Sens. Actuators A 114(2–3), 244–249 (2004).
- 115 Wireless biomechanical power harvesting via flexible magnetostrictive ribbons. Energy Environ. Sci. 7(7), 2243–2249 (2014).
- 116 . Recent progress in micro-supercapacitors with in-plane interdigital electrode architecture. Small 13(45), 1–10 (2017).
- 117 Ultralight cut-paper-based self-charging power unit for self-powered portable electronic and medical systems. ACS Nano 11(5), 4475–4482 (2017).
- 118 . Piezoelectric-driven self-charging supercapacitor power cell. ACS Nano 9(4), 4337–4345 (2015).
- 119 . Materials for electrochemical capacitors. Nat. Mater. 7(11), 845 (2008).
- 120 . Wireless power system for charge supercapacitors as power sources for implantable devices. Presented at: 2015 IEEE PELS Workshop on Emerging Technologies: Wireless Power (WoW). Daejeon, South Korea, 5–6 June (2015).
- 121 . A flexible super-capacitive solid-state power supply for miniature implantable medical devices. Biomed. Microdevices 15(6), 973–983 (2013).
- 122 . A wirelessly powered and controlled device for optical neural control of freely-behaving animals. J. Neural Eng. 8(4), 046021 (2011).
- 123 . Electrochemical capacitors: mechanism, materials, systems, characterization and applications. Chem. Soc. Rev. 45(21), 5925–5950 (2016).
- 124 . Microbattery technologies for miniaturized implantable medical devices. Curr. Pharm. Biotechnol. 11(4), 404–410 (2010).
- 125 . High-power lithium ion microbatteries from interdigitated three-dimensional bicontinuous nanoporous electrodes. Nat. Commun. 4, 1732 (2013).
- 126 On-chip and freestanding elastic carbon films for micro-supercapacitors. Science 351(6274), 691–695 (2016).
- 127 . High-resolution on-chip supercapacitors with ultra-high scan rate ability. J. Mater. Chem. A 2(20), 7170–7174 (2014).
- 128 . Are tomorrow's micro-supercapacitors hidden in a forest of silicon nanotrees? J. Power Sources 269, 740–746 (2014).
- 129 . 3D RuO2 microsupercapacitors with remarkable areal energy. Adv. Mater. 27(42), 6625–6629 (2015).
- 130 . Wafer-level fabrication process for fully encapsulated micro-supercapacitors with high specific energy. Microsystem Technol. 18(4), 467–473 (2012).
- 131 Hydrous RuO2/carbon nanowalls hierarchical structures for all-solid-state ultrahigh-energy-density micro-supercapacitors. Nano Energy 10, 288–294 (2014).
- 132 . Microsupercapacitors as miniaturized energy-storage components for on-chip electronics. Nat. Nanotechnol. 12(1), 7 (2017).
- 133 . Design of architectures and materials in in-plane micro-supercapacitors: current status and future challenges. Adv. Mater. 29(5), 1–19 (2017).
- 134 . Capacitive energy storage in micro-scale devices: recent advances in design and fabrication of micro-supercapacitors. Energy Environ. Sci. 7(3), 867–884 (2014).
- 135 . A review of on-chip micro supercapacitors for integrated self-powering systems. J. Microelectromechanical Syst. 26(5), 949–965 (2017).
- 136 . Proton-conducting polymer electrolytes and their applications in solid supercapacitors: a review. RSC Adv. 4(62), 33091–33113 (2014).
- 137 . Ionogels, ionic liquid based hybrid materials. Chem. Soc. Rev. 40(2), 907–925 (2011).
- 138 . Ultrasmall integrated 3D micro-supercapacitors solve energy storage for miniature devices. Adv. Energy Mater. 4(7), 1–7 (2014). • Demonstrates the integration of a supercapacitor in a microdevice.
- 139 Bioresorbable silicon electronic sensors for the brain. Nature 530(7588), 71–76 (2016).