Markers of rejection of a lung allograft: state of the art
Abstract
Chronic lung allograft dysfunction (CLAD) affects approximately 50% of all lung transplant recipients by 5 post-operative years and is the leading cause of death in lung transplant recipients. Early CLAD diagnosis or ideally prediction of CLAD is essential to enable early intervention before significant lung injury occurs. New technologies have emerged to facilitate biomarker discovery, including epigenetic modification and single-cell RNA sequencing. This review examines new and existing technologies for biomarker discovery and the current state of research on biomarkers for identifying lung transplant rejection.
Lung transplantation improves the life expectancy and quality of life in patients with progressive lung disease such as chronic obstructive pulmonary disease (COPD), pulmonary fibrosis (PF) and cystic fibrosis (CF). However, despite increased organ procurement, improved surgical techniques and perioperative care since the early lung transplantation era of the 1980s, long-term survival trends (greater than 5 years) remain similar. Transplanted lungs remain at risk of chronic rejection, also called chronic lung allograft dysfunction (CLAD) (reviewed in [1]). CLAD affects approximately 50% of all lung transplant recipients by 5 post-operative years. With a survival rate of approximately 50% at 2.5 years post-diagnosis, it remains the leading cause of death in lung transplant recipients, reducing quality of life and increasing economic burden [2].
CLAD is defined as a persistent, irreversible drop in lung function measured as forced expiratory volume in 1 s (FEV1) or forced vital capacity (FVC) to 20% or more from the post-transplant baseline after exclusion of alternate causes for reduced graft function [3]. CLAD was initially thought to result from T-cell injury; however, a growing body of evidence suggests that multiple arms of the immune system are involved, including monocytes, neutrophils and autoantibodies (reviewed in [1]). Other events such as gastroesophageal reflux, infection, reperfusion injury and air pollution can impose a higher risk of developing CLAD (reviewed in [1]). Such persistent insult activates or inflames the epithelium, combining with impaired wound healing and leading to lung fibrosis and decline in lung function (reviewed in [1]).
Heterogeneity of CLAD
Distinct phenotypes of CLAD occur with variable pathology, diverse airway neutrophilia, fibrosis, histological features and responsiveness to therapy. The recognized phenotypes are bronchiolitis obliterans syndrome (BOS) and restrictive allograft syndrome (RAS) [3]. BOS is the most common form of CLAD and is seen in 50% of patients within 5 years of transplant (reviewed in [4]). BOS is defined by a persistent 20% drop in FEV1 and an obstructive pattern in pulmonary function tests (PFTs) with no or minimal evidence of parenchymal fibrosis [5]. RAS is less common but has a worse prognosis and is classified by a 20% drop in FEV1 and reduced FVC and total lung capacity, but it has a restrictive pattern in PFTs and opacities in chest CT scans and x-rays [6]. Complicating diagnosis, CLAD may arise in mixed forms with similar mechanisms of disease progression. Reliable biomarkers that identify these phenotypes are yet to be identified (reviewed in [7,8]).
The need for CLAD biomarkers
Persistent episodes of acute rejection are a risk factor for CLAD and currently diagnosed by decline in lung function and perivascular cellular infiltrates present in transbronchial biopsies (TBBs). Bronchoalveolar lavage (BAL) is also an important tool to monitor rejection. Cellular composition (increased lymphocyte to macrophage ratio) is diagnostic of rejection, and culture of supernatant or PCR can identify infectious agents (bacteria, viruses, fungi) that impact lung function. Although biopsies are routinely performed, the procedure has only 30% sensitivity to identify chronic rejection, is invasive, imposes procedural risks (such as bleeding and pneumothorax), requires multiple sampling to reflect overall lung pathology and is subject to variability due to observational bias. Therefore, there is a need to identify biomarkers of cellular rejection preceding CLAD to stratify patients according to risk and facilitate personalized treatment options based on risk levels. Several biomarkers associated with CLAD have been evaluated, but implementation into diagnostics has not yet eventuated due to poor specificity or sensitivity, as well as failure to detect early-stage disease (reviewed in [7,8]). Early CLAD diagnosis or ideally prediction of CLAD is essential to enable early intervention before significant lung injury occurs. This review examines the current state of research on biomarkers for monitoring or detecting lung transplant rejection.
Biomarkers in blood
As blood is easily accessible, several studies have examined blood biomarkers and their association with lung rejection and CLAD. Although blood provides insight into systemic changes in the body, blood biomarkers may not necessarily reflect the lung microenvironment. This is an important consideration in lung transplantation, in which monitoring the underlying systemic allo-immune response to the transplanted lung is required to detect low-grade rejection. Several studies have investigated various constituents of blood, including cells [9–19], cytokines and chemokines [20–23], in search of biomarkers for chronic rejection in lung transplant patients.
Of the immune cells found in blood, Tregs are the most intensively studied cell type in lung transplant rejection due to their key role in immune regulation. Multiple Treg subpopulations have been studied in lung transplantation and are distinguished based on the presence of markers such as CD25 and FoxP3. Several studies report increased Tregs in patients experiencing CLAD compared with stable patients [10,12,14,15], whereas other studies show a decrease in Tregs [13,24]. Other than Tregs, Budding et al. investigated variations in other cell types in the blood of lung transplant recipients with and without BOS [16]. In their study, CD8+ T lymphocytes, B lymphocytes and monocytes were decreased, and natural killer cells increased, in BOS patients. Eosinophils in blood were increased in patients developing CLAD [17,18]. Coiffard found blood neutrophils elevated in patients with CLAD [19]. Bergantini et al. also showed reduced B lymphocytes during BOS [25]. Table 1 summarizes studies investigating immune cells in blood in relation to rejection and CLAD.
Cell type | Patient observation | Sample size | Ref. |
---|---|---|---|
Treg (CD4+CD25+) | Decreased in BOS | Stable = 10, BOS = 11, healthy = 7 | [9] |
Treg (CD4+CD25+CD69-) | Increased in evolving OB compared with healthy LTR | AR = 7, stable OB = 7, evolving OB = 13 | [10] |
Treg (CD4+FoxP3+) | No change in Tregs | Stable = 14, BOS = 6 | [11] |
Treg (CD4+CD25+CD127-, CD4+CD25+FoxP3+, CD4+CD25+IL2+) | Increasing frequencies with a protective role against developing early CLAD | CLAD = 31, stable = 107 | [12] |
Treg (CD4+CD25+CD127-) | Decreased in CLAD | n = 137 | [13] |
Increased Tregs associated with better freedom of CLAD | n = 724 (66% CLAD-free) | [14] | |
Decreased during acute rejection | AR = 6, BOS = 6, stable = 4 | [25] | |
Treg (CD4+CD25+FoxP3+) | Increased in BOS patients at 1–6 months post-Tx | Stable LTR = 50, BOS = 32 | [15] |
CD8+ lymphocytes | Decreased in BOS | BOS = 11, non-BOS = 39 | [16] |
NKT cells | Increased BOS | BOS = 11, non-BOS = 39 | [16] |
Decreased in during AR and BOS | AR = 6, BOS = 6, stable = 4 | [25] | |
B lymphocytes | Decreased in BOS | BOS = 11, non-BOS = 39 | [16] |
Regulatory B cells (CD19+CD24+CD38+, CD19+CD24+CD27+) | Decreased in AR | AR = 6, BOS = 6, stable = 4 | [25] |
Monocytes | Decreased in BOS | BOS = 11, non-BOS = 39 | [16] |
Eosinophils | Increased in CLAD | n = 319 | [17] |
Increased in patients with worse survival (CLAD) | n = 376 | [18] | |
Neutrophils | Increased in CLAD | Stable = 80, CLAD = 23 | [19] |
A number of pro-inflammatory cytokines and chemokines involved in cell recruitment and activation correlate with lung transplant rejection: IL-1 and IL-12 increased in BOS [20]; IFN-γ and MCP-1 increased with lung injury associated with BOS/CLAD [20,26]; the inflammatory marker CRP was elevated in the blood of patients with BOS [23]; and the anti-inflammatory cytokines IL-10 and IL-4 were decreased in patients with BOS [20,21].
Biomarkers in BAL
BAL is a routine technique performed at the time of bronchoscopy to collect respiratory secretions for diagnosis and research purposes [27]. Compared with TBB, BAL is safer and samples a relatively larger area of the lung, enabling repeated access to transplanted lungs [28]. Despite its lower invasiveness, the procedure itself has several limitations: different procedures in collecting BAL and sampling size result in variability [27], and the composition and volume of the instilled fluid together with dilution factors related to the BAL technique, alveolar permeability at the time of sampling and the contamination of BAL with blood affect the BAL fluid composition [29]. In order to correct for dilution factors in BAL fluid, the coefficient of urea-in-plasma/urea-in-BAL is used for standardization [30]; however, infections can increase urea in the lung and plasma, leading to inaccurate quantitation [31]. To minimize inaccuracies, The International Society for Heart and Lung Transplantation has taken steps to standardize BAL techniques among centers [27], enabling the comparison of findings across studies. Notwithstanding these limitations, BAL fluid remains an important window into the biomarker status of the lung following lung transplantation.
Like blood, cells collected from BAL fluid have been analyzed to investigate their relationship to lung transplant rejection (summarized in Table 2). Tregs have been shown to decrease in the BAL of patients experiencing CLAD [10,11,25,32]. Multiple studies also show that there is an elevation of total lymphocytes in the BAL in CLAD patients compared with that of stable lung transplant recipients [33–35]. Studies report discordant results with respect to macrophages in BAL with increasing proportions in CLAD (RAS and BOS) [33] or a decrease in proportion in BOS patients [34]. Multiple studies have reported an increase in neutrophils in the BAL with CLAD [33–36]. Furthermore, increased eosinophil counts in patients developing CLAD [17] and decreased Bregs during BOS have been reported [25].
Cell type | Observation | Sample size | Ref. |
---|---|---|---|
Treg (CD4+CD25+CD69-) | Increased in stable OB and evolving OB compared with healthy LTR | AR = 7, stable OB = 7, evolving OB = 13 | [10] |
Treg (CD4+FoxP3+) | Decreased in LTR with BOS | Stable = 14, BOS = 6 | [11] |
Treg (CD4+CD25+FoxP3+CCR7+) | Increased in patients with no BOS | No BOS = 34, BOS = 13 | [32] |
Treg (CD25+CD127-) | Decreased in BOS | AR = 6, BOS = 6, stable = 4 | [25] |
Th1 (CD4+CD45-CCR6-CXCR3+CCR4-) | Increased in BOS | AR = 6, BOS = 6, stable = 4 | [25] |
Th2 (CD4+CD45-CCR6-CXCR3-CCR4+) | Increased in AR | AR = 6, BOS = 6, stable = 4 | [25] |
Th17 (CD4+CD45-CCR6+CXCR3-CCR4+) | Increased in BOS | AR = 6, BOS = 6, stable = 4 | [25] |
Total lymphocytes | Increased in CLAD (RAS and BOS) | Stable = 14, BOS = 15, RAS = 15 | [33] |
Increased in BOS | Stable = 32, infection = 11, BOS = 36, AR = 43 | [34] | |
Increased before CLAD | CLAD = 47, no CLAD = 93 | [35] | |
B lymphocytes | Increased in BOS | AR = 6, BOS = 6, stable = 4 | [25] |
Regulatory B cells (CD19+CD1d+CD5+) | Decreased in AR and BOS | AR = 6, BOS = 6, stable = 4 | [25] |
Neutrophils | Increased in BOS | BOS = 16, no BOS = 47 | [36] |
Increased in CLAD (RAS and BOS) | Stable = 14, BOS = 15, RAS = 15 | [33] | |
Increased in BOS | Stable = 32, infection = 11, BOS = 36, AR = 43 | [34] | |
Increased before CLAD | CLAD = 47, no CLAD = 93 | [35] | |
Increased in BOS and RAS patients compared with stable | Stable = 29, BOS = 15, RAS = 7 | [37] | |
Eosinophils | Increased in CLAD | n = 319 | [17] |
Cytokines and chemokines in BAL fluid have been analyzed in relation to lung transplant rejection (summarized in Table 3). A consistent finding is that pro-inflammatory markers correlate with rejection: IL-1 and IL-6 are increased in BAL from patients with BOS [38–40]; however, no differences were observed in IL-6 between acute rejection and BOS [41]. Multiple studies show IL-8 is increased in BAL from patients with BOS compared with stable patients [38,42,43]. Both pro-inflammatory cytokines, IFN-γ and TNF-α and fibrotic cytokines, TGF-β, are elevated in BAL of BOS [40,42,44].
Cytokine | Role | Compartment | Observation with respect to lung injury/rejection | Sample size | Ref. |
---|---|---|---|---|---|
IL-1 | Multifunctional pro-inflammatory cytokine | BAL | Increased | n = 20 | [40] |
BAL | Increased | Stable = 13, CLAD = 42 | [39] | ||
Serum | Increased | BOS = 31, no BOS = 31 | [20] | ||
BAL | increased | Stable = 20, non-neutrophilic BOS = 20, neutrophilic BOS = 17, RAS = 20 | [38] | ||
IL-4 | Anti-inflammatory cytokine released by Th2 T cells, basophils and mast cells | Serum | Decreased | BOS = 10, no BOS = 10 | [21] |
IL-6 | Major pro-inflammatory cytokine involved in vascular remodeling and pulmonary hypertension in the lungs | BAL | Increased | Stable = 20, non-neutrophilic BOS = 20, neutrophilic BOS = 17, RAS = 20 | [38] |
BAL | Increased | Stable = 13, CLAD = 42 | [39] | ||
BAL | No change | AR = 37, BOS = 48 | [41] | ||
IL-8 | Chemotactic factor for neutrophils secreted by macrophages and respiratory epithelial cells; associated with lymphocytic airway inflammation and neutrophilic reversible allograft dysfunction | BAL | No change | AR = 37, BOS = 48 | [41] |
BAL | Increased | Stable = 20, non-neutrophilic BOS = 20, neutrophilic BOS = 17, RAS = 20 | [38] | ||
BAL | Increased | Stable = 13, BOS = 8 | [43] | ||
BAL | Increased | BOS = 10, no BOS = 19 | [42] | ||
BAL | No change | Stable = 17, AR = 9 | [58] | ||
IL-10 | Anti-inflammatory cytokine secreted by Th2 cells and regulatory T cells; dampens the immune response by inhibition of T cells, monocytes and macrophages | BAL | Increased | n = 20 | [40] |
Blood | Decreased | BOS = 31, no BOS = 31 | [20] | ||
IL-12 | Produced by dendritic cells, macrophages and monocytes; important regulator of Th1 responses and promotes expansion and survival of activated T cells and NK cells. | Blood | Increased | BOS = 31, no BOS = 31 | [20] |
BAL | Decreased | n = 44 | [9] | ||
IL-17 | Pro-inflammatory cytokine secreted by CD4+ T cells with an important role in mobilization of neutrophils via induction of IL-8 | BAL | Increased | Stable = 17, AR = 9 | [58] |
BAL | Increased | Stable = 72, BOS = 29 | [59] | ||
CXCL10 | IFN-γ-induced chemokine promoting directional migration of activated T cells during inflammation | Biopsy tissue | Increased | n = 24 | [60] |
CXCL9 | IFN-γ-induced chemokine inducing chemotaxis, differentiation and proliferation of immune cells and tissue extravasation | BAL | Increased | Stable = 234, CLAD = 207 | [61] |
IFN-γ | Pro-inflammatory cytokine secreted by Th1 cells and NK cells; enhances macrophages to degrade pathogens and inhibits Th2 cell differentiation; NK cell activity and B-cell regulation | BAL | Increased | n = 20 | [40] |
Blood | No change | Stable = 31, AR = 10 | [62] | ||
TNF-α | Pro-inflammatory cytokine secreted by macrophages, T cells and NK cells | BAL | Increased | n = 20 | [40] |
BAL | Increased | Stable = 13, CLAD = 42 | [39] | ||
TGF-β | Anti-inflammatory cytokine with strong immune-regulatory properties, including profibrotic role in the lung | BAL | Increased | n = 70 | [44] |
BAL | Increased | BOS = 10, no BOS = 19 | [42] | ||
MCP-1 | Chemokine regulating migration and infiltration of monocytes/macrophages | Serum | Increased | BOS = 31, no BOS = 31 | [20] |
BAL | Increased | Stable = 13, BOS = 8 | [43] | ||
CRP | Serum pentraxin and an acute phase reactant synthesized in response to inflammation, infection and tissue damage; mediates activation of monocytes/macrophages; accumulates selectively in sites of inflammation | BAL | Increased | Stable = 67, BOS = 54 | [23] |
Blood | Increased | Stable = 67, BOS = 54 | [23] |
Although these studies highlight CLAD-specific biomarkers, to date no point of care tests using blood or BAL biomarkers have been developed to monitor lung transplant rejection.
Epigenetic markers
DNA methylation is a reversible gene regulatory process that is modifiable by aging, environmental influences and lifestyle factors such as smoking or diet. In particular, oxidative stress can induce promoter CpG methylation by recruiting MECP2 and DNA methyltransferases (reviewed in [45]). DNA methylation, like telomeres, can provide an approximate age of cells (‘epigenetic clock’) (reviewed in [46]). The difference between DNA methylation predicted age (DNAm age) and chronological age is the accelerated epigenetic age and is associated with several diseases [47]. DNAm age provides a reliable estimate of cell age at different time points of life. DNAm age has been successfully used in the identification of high-risk individuals prone to accelerated aging in a number of diseases such as cancer, diabetes, cardiovascular diseases, dementia and post-traumatic stress syndrome (PTSD) (reviewed in [46]). Recent reports show that idiopathic pulmonary fibrosis (IPF) lungs have increased DNA methylation status in gene promoters [48,49]. Differential DNA methylation may provide a potential novel epigenetic biomarker for smoking-related lung diseases, including COPD and lung cancer (reviewed in [50]). A recent epigenome-wide association study identified 59 differentially methylated regions (DMRs) in cord blood associated with childhood lung function, as well as 15% associated with COPD in adults [51]. Another study of peripheral blood mononuclear cell (PBMC) methylation using Illumina Infinium® MethylationEPIC (EPIC) arrays identified 28 differentially methylated sites, with 26 associated with lung function and two associated with COPD (cg18181703 in SOCS3 and cg18608055 in SBNO2) [52]. In transplant patients, epigenetic markers responsible for the regulation and expression of genes important in rejection have not been identified. Genome-wide epigenetic analysis of stable and CLAD patients is needed to identify biomarkers for rejection. A handful of studies have examined epigenetic biomarkers predicting rejection episodes in heart (reviewed in [53]) and kidney transplantation (reviewed in [54]) but, to the authors' knowledge, epigenetic markers in lung transplantation are yet to be identified. DNA methylation of genes activated during rejection episodes could provide valuable information on the underlying immune and other cell pathways that are active and may predict post-transplant complications such as ischemia injury and rejection.
Gene-expression markers
Because acute cellular rejection (ACR) pathologies have been associated with increased CLAD risk, methods to detect cellular rejection are important for clinicians to monitor and treat rejection episodes prior to lung function decline. The gold standard continues to be TBB and BAL using histological scoring and lymphocyte counts, respectively, to approximate T-cell numbers [55]. However, histological assessment of T-cell-mediated rejection is difficult to reproduce, and failure to diagnose rejection can lead to insufficient or excessive immunosuppression [56]. Thus, there has been an effort to identify biomarkers of cellular rejection. Lung cell profiles translate to unique transcriptome signatures, providing potential biomarkers to aberrant pathways in lung transplant rejection. Methods to measure differential gene expression in cells and tissue include RNA micro-array profiling and RNA sequencing, which offers increased dynamic range and enhanced the sensitivity/detection of low abundance transcript. Both approaches have led to the identification of biomarkers associated with rejection and the use of PCR-based assays for specificity and sensitivity in detecting rejection. An early study using micro-array compared 32 TBB samples from patients whose concurrent histology showed acute rejection (n = 14) or no rejection (n = 18) and identified over 100 genes differentially expressed [57]. Consistent with the paradigm of ACR and CLAD pathobiology of activated T cells leading to cytotoxicity, causing allograft injury and CLAD, genes enriched in T-cell function, cytotoxic CD8 activity and granulocyte degranulation were identified. Translating this to a potential diagnostic qPCR test, ten of the 52 genes showed increased expression in samples from patients with acute rejection [57]. Although these studies present promising evidence showing a T-cell molecular signature in rejection, no diagnostic test has replaced TBB and BAL.
In another study, of RNA profiling of blood cells from lung transplant recipients, Danger et al. identified three downregulated genes, POU2AF1, TCL1A and BLK, that predicted BOS 6 months before diagnosis compared with stable lung transplant recipients. Levels of gene expression stratified patients in survival analysis based on BOS risk [63]. A similar study identified 40 genes that were differentially expressed in BAL cells between CLAD and CLAD-free patients. Genes were related to recruitment, retention, activation and proliferation of cytotoxic lymphocytes such as CD8+ T cells and natural killer cells, consistent with episodes of repeat acute cellular rejection and CLAD [28]. Meta-analysis of datasets from 236 graft biopsy samples (kidney, heart, lung and liver) identified a common rejection module (CRM) consisting of 11 genes, overexpressed in acute rejection, across all transplanted organs [64]. Applied to patient biopsies 6 months post-lung transplant, CRM was upregulated in four TBB rejection positive samples compared with ten TBBs without rejection. A difference was also observed with CLAD phenotypes, with CRM higher in RAS explanted lungs compared with donor lungs. Two genes in the CRM (ISG20 and CXCL9) were upregulated in RAS or BOS lungs compared with donor lungs [65]. Although promising, the CRM was only applicable to TBB and not BAL cells, limiting the applicability of the assay.
An alternate approach to PCR is NanoString, a clinical platform superior to TBB and BAL. NanoString technology has several benefits over other methods, including no requirement for amplification, high sensitivity and specificity. This approach has potential for an automated platform for routine diagnostic workflows across pathology laboratories [66]. Banff Molecular Diagnostics Working Group has taken the initiative to pioneer the Banff Human Organ Transplant (B-HOT) panel, using NanoString technology to include validated genes from previous studies on kidney, heart, lung and liver allograft biopsies. The panel consists of 770 genes associated with organ rejection, tissue damage and immune responses. In practice, this panel would be useful to detect and monitor rejection with work ongoing to validate the accuracy and sensitivity of the panel [66]. This approach is promising, as both rejection-associated tissue damage and immune reactivity are measured simultaneously.
With advances in technologies, whole transcriptomic analysis of cells has become affordable and rapid and is becoming an essential tool in biomarker discovery. Generating a vast amount of useful data in a short time, genes co-regulated with disease are identifiable and pathways assembled linked to disease. In transplantation, transcriptomics will identify molecular mediators and mechanisms of tissue damage leading to rejection,, including genes and pathways predictive of pathological changes in the allograft and likelihood of graft outcome, for example, time to CLAD. Cost–effectiveness and reproducibility across multiple centers make transcriptomics a potential game changer for the diagnosis, prognosis and management of transplant patients.
In addition to gene expression, single-nucleotide polymorphisms (SNPs) associate with post-lung transplant mortality. Notably, donor SNPs in human leukocyte antigen (HLA) G gene (HLA-G*01:04 haplotype) increase expression of HLA-G and exacerbate CLAD risk and mortality [67]. Soluble HLA-G concentrations in BAL correlate with the number of acute rejection episodes in the first 12 months after lung transplantation and may be a biomarker of rejection [68,69]. Similarly, HLA-E*01:03 allele is associated with the development of CLAD [70]. Other SNPs associated with CLAD or mortality include Fc Gamma Receptor IIa [71,72], IL-17R and IL-23R [73] and Dectin-1 (CLEC7A gene) [74]. A common theme of these SNPs is immune modulation, which impacts graft survival and tolerance. SNP typing of patients or measurement of impacted protein biomarkers will likely be useful in the stratification of patients for risk and the implementation of personalized targeted therapeutics.
miRNA markers
miRNAs are non-coding, single-stranded RNA 22–25 nucleotides in length, with the ability to repress translation and/or degrade mRNA as a post-transcriptional regulatory point in gene expression by binding to the 3′ UTR of target genes. Their origin is unclear but they may arise from damaged or apoptotic cells. In lung transplantation, miRNA expression profiling of PBMCs and BAL in stable lung transplant recipients, recipients with donor-specific antibodies (DSA) and no BOS and recipients with DSA and BOS found different miRNA profiles with respect to differentially expressed genes in signaling pathways of B cells, Toll-like receptors, TGF-β, chemokine receptors and cytokine–cytokine interactions [75]. Interestingly, in BOS, two miRNAs (miR-10a and miR-133b) were differentially expressed in both BAL and blood. miR-10a is involved in the differentiation of hematopoietic cells specifically in Treg stability and functioning [76] and miR-133b co-regulated with IL-17 production [77]. Variations in the expression of these miRNAs preceded BOS development and most of the differently expressed miRNA was involved in vital cellular processes such as cell cycle signaling pathways [75]. These findings highlight a likely role of miRNA in the development of BOS and the utility of miRNA as a biomarker for early detection of rejection. However, studies in larger cohorts are required to validate the accuracy and reproducibility of these results.
cfDNA markers
cfDNA is DNA released into the bloodstream by dying cells and is a direct measure of organ injury. Increased cfDNA occurs with sepsis, trauma and cancer (reviewed in [78]). Importantly in transplantation, genetic disparity between the donor and recipient enables discrimination and quantification of donor-derived cfDNA (ddcfDNA) as a marker of allograft injury [79]. Due to its short half-life, ddcfDNA can provide a time-dependent resolution to understanding ongoing immunomodulatory events occurring at the time of rejection. This has been demonstrated in kidney, heart and liver transplantation (reviewed in [80]).
A handful of studies have investigated the possibility of using ddcfDNA in lung transplantation to detect, assess and monitor rejection. A proof-of-concept study by Agbor-Enoh et al. showed that the average percentage of ddcfDNA in plasma strongly associates with the development of allograft failure and all-cause mortality in lung transplantation. Importantly, this study showed the average percentage of ddcfDNA detected clinically silent episodes of acute rejections and enabled stratification of the patient cohort based on the risk of subsequent allograft injury [81]. An independent study reported that cfDNA in BAL was elevated in patients with BOS compared with stable patients and combining cfDNA and CXCL10 quantitation in BAL facilitated the stratification of BOS, RAS and stable phenotypes [82]. These findings reinforce the idea that multiple biomarkers may offer the most sensitive and accurate approach to diagnosing and predicting lung transplant rejection.
There are, however, limitations to measuring ddcfDNA, including the need to sequence and SNP type both donor and recipient cells. Furthermore, reliance on the percentage of ddcfDNA may be influenced by changes in recipient cfDNA levels due to infection and granulocyte cell death (reviewed in [83]).
An alternative approach to measuring total ddcfDNA is to use methylation-specific PCR to quantitate only transplant rejection-associated cell cfDNA and use this as a biomarker of CLAD risk. Methylation of DNA-encoding genes and transcriptional enhancers is essential to silencing gene expression. Conversely, demethylation, which can occur throughout life, enables transcription factors to bind DNA and induce gene expression. Thus, methylation patterns are ideal for identifying cell types in mixed populations, as they reflect the transcriptome, function and ontogeny of cells [84]. Measuring DNA methylation of cfDNA for the identification of new biomarkers in lung transplant rejection may offer a sensitive method of measuring donor lung damage preceding CLAD.
Biomarkers of allo-immune & auto-immune responses
Allo-immune responses impact CLAD with mismatched HLAs increasing risk [85–90]. HLA mismatching of donor and recipient results in anti-HLA donor-specific antibodies (DSAs). Pre-transplant DSAs are a prominent risk factor for CLAD and mortality [91,92] and, in organ allocation, potentially reactive HLA mismatches in the donors via virtual crossmatching of the recipient are avoided [93,94]. Similarly, post-transplant DSAs increase CLAD [91,95–103] and DSA measurements are routinely used in recipient follow-up and risk stratification [104]. The combined use of HLA antibodies and CXCL9 levels has recently enabled the identification of recipients at high risk of CLAD [105]. Interestingly, lung transplant recipients with antibodies to the ubiquitous self-antigens, collagen V (ColV) and K-α 1 tubulin (Kα1T) have increased the risk of chronic rejection [106–110].
Many of the previously mentioned immune modulatory biomarkers are present in circulating exosomes. Exosomes are released from donor and recipient cells and contain biomarkers relevant to solid organ transplantation tolerance and rejection [111,112]. In lung transplantation, mismatched donor HLA and lung antigens, including ColV and Kα1T; immune modulatory molecules, including miRNAs responsible for inducing inflammation; TH-17 differentiation; and endothelial activation have been identified in exosomes during acute or chronic rejection [108,113–118]. These and other biomarkers are reflective of allo- and auto-immune modulation, and their abundance may reveal lung transplant recipients with a heightened risk of CLAD. Combined with additional novel biomarkers, including lung microbiome characteristics [119] and nutritional assessment scores such as prognostic nutrition index (PNI) [120], the accuracy of CLAD prediction may be improved. Combinatorial biomarker assessment would increase pre-operative risk management in lung transplantation and efficient organ procurement when used together with recently developed virtual crossmatch-based strategies [94]. Utilization of these novel approaches with multi-omic profiling [121] would aid in the effective prediction of CLAD and lung allograft outcome.
Biomarkers of biological aging
Aging in general is the progressive decline in homeostasis after the reproductive phase of life, leading to increasing risk of disease or death. Though biological aging is often linked to chronological aging, it can also occur earlier in life due to malfunctions in organ or cell maintenance and repair (reviewed in [122]). Such accelerated biological aging has been emphasized as a strong risk factor for a number of human diseases such as cardiovascular diseases, metabolic diseases, neurological diseases and cancer (reviewed in [123]). Markers of biological aging may be reliable clinical biomarkers to detect, predict and monitor age-related diseases. Markers of aging include cellular senescence, genomic instability, telomere attrition and mitochondrial dysfunction (reviewed in [123]). Lung function declines with age [124] and the correlation of lung capacity with a biomarker of accelerated aging of the lung may be a good predictor of lung transplant rejection.
Telomere length is a strong predictor of biological aging in cells. Telomeres are nucleotide repeats of TTAGGG at the ends of chromosomes that provide protection during cell replication. Telomeres shorten over time and extreme shortening can lead to cell senescence via the activation of DNA damaging pathways. Telomere length differs inversely with age and short telomeres trigger cell senescence. Several syndromes have been identified with accelerated telomere shortening [125], and inherited and sporadic mutations in the telomerase genes are associated with early aging [126]. These genes include TERT, TERC, PARN, RTEL1 and NAF1 and are associated with familial PF, but not with sporadic and non-familial PF [127]. PF is also known to result from immune rejection leading to CLAD [5], and telomere length may be useful as a biomarker of lung transplant-associated PF. Transplantation offers a unique opportunity to study PF outcome of mismatched telomere length in donor and recipient cells, in turn informing which compartment, immune or parenchymal, drives transplant-associated PF. In renal and stem cell transplantation, telomere shortening correlates with poor graft survival [128,129].
In lung transplantation, a preliminary study reported shorter recipient lung telomeres associated with a decreased rate of acute cellular rejection, but neither the telomere length of the donor nor that of the recipient was associated with survival. Both Donor lymphocyte and recipient lung tissue telomere lengths were inversely related to age [130]. Shorter telomere length was associated with reduced post-transplant survival, incidence of CLAD, shorter time to CLAD and increased infection in a further study [131]. A recent study found that short telomeres early post-transplant were associated with leukopenia and decreased CLAD-free survival [132]. Another study, by Faust et al., reported telomere shortening in airway epithelial cells, which was associated with CLAD. The study examined 175 lung transplant recipients and measured the telomere lengths of both donors and recipients using qPCR and QFISH assays. Shorter telomere length was associated with an increased risk of CLAD or death. Short PBMC telomere length was associated with significantly worse CLAD-free survival and increased the occurrence of post-transplant leukopenia [133]. In contrast, Mackintosh et al. recently showed that short telomeres strongly associate with donor age and smoking history but show no association with the future incidence of CLAD [134]. Cytomegalovirus (CMV) infection in lung transplant recipients augments the shortening of telomeres, likely as a result of increased cell turnover due to infection, suggesting that telomerase activity may be important in the regeneration and healing of airway epithelia in response to lung injury [135]. Finally, variants of recipient TERT, RTEL1 and PARN genes associate with shorter time to CLAD and explicitly restrictive CLAD (R-CLAD) [136]. These studies suggest that shorter telomeres can directly correlate with negative outcomes in lung transplantation.
Future biomarkers & single-cell RNA sequencing
A major limitation of the clinical use of biomarkers for diagnosis is the source of samples. Both blood and BAL have complex cell populations and separation of cells is costly, time consuming and not always possible. Therefore, bulk samples are the main source for biomarker testing for practical purposes. This poses challenges, as biomarkers may be present in small populations of cells that vary over time and the testing of bulk RNA may lead to poor reproducibility. In an effort to understand cellular heterogeneity and cell-specific pathways in health and disease, new techniques to analyze the transcriptome of individual cells in mixed populations are becoming available. single-cell RNA sequencing is becoming the preferred method. This technology partitions cells, adds a genetic barcode and following bulk DNA sequencing and alignment to a reference genome, the transcriptome of each cell is determined. Downstream bioinformatics generates count tables of gene expression at individual cell level. Applying dimensional reduction and machine learning, cells are clustered by gene signature and assigned a cell type based on their gene-expression profile.
Applied to healthy lung tissue, which has previously been estimated to have 40 different cell types [137], single-cell RNA sequencing was able to detect 58 cell populations [138]. In the context of lung disease, single-cell RNA sequencing has identified changes in cells from unused donor lungs compared with explant lungs from patients with PF, including IPF and interstitial lung disease (ILD). Recent findings include the discovery of distinct epithelial cell populations, including an aberrant basaloid epithelial cell increased in fibrotic lungs [139–141], increased goblet cells and Th2 cells in asthma [142] and identification of monocyte-derived macrophages with fibrotic transcriptome enriched in ILD [140,143,144]. Clearly, for diagnosis and patient monitoring, explant tissue is not possible, so single-cell RNA sequencing methods need to adapt to TBB, BAL and blood. Reyfman et al. demonstrated that cryobiopsies can be analyzed at the single-cell level [143], and Morse showed macrophage populations in BAL of healthy patients [144]. However, these studies were only exploratory demonstrating feasibility, and the cell types, transcriptome and relationship to disease remain unexplored, requiring larger comparative studies of healthy and diseased samples. In lung transplantation, single-cell RNA sequencing combined with male- and female-specific markers has been used to assess the engraftment of cells in sex-mismatched transplant recipients [145]. Although only four BAL samples were analyzed, and patients were a year or more post-transplant, a high level of replacement of donor immune cells was observed with the majority of lung cells being of recipient origin, confirming a higher-than-expected turnover of alveolar macrophages in the transplanted lung. Future studies particularly in the first 6 months following transplantation are needed to fully characterise cellular changes in donor and recipient cells and their relationship to primary graft dysfunction, an important risk factor for developing CLAD. A recent study examined BAL from a transplant recipient with CLAD and demonstrated that monocyte-derived macrophages, of recipient origin, expressed profibrotic genes, including autotaxin (ENNP2), the main enzyme able to generate LPA, implicated in fibroblastic recruitment and differentiation [146]. In the small cohort of transplant patients, ENPP2 expression in BAL cells, as assessed by PCR, correlated with time to CLAD [146], demonstrating the utility of single-cell RNA sequencing as a new tool for biomarker discovery in cells from BAL, routinely collected from lung transplant recipients for surveillance bronchoscopy. Surprisingly, to date, no studies have reported cell types and transcriptome of blood cells in lung transplant patients by single-cell RNA sequencing. Considering the large number of studies showing blood cell changes and association with lung transplant rejection (Table 1), single cell analysis should provide an opportunity to analyze all these cell types and their active pathways simultaneously, providing unparalleled insight into blood cell dynamics. Future work will hopefully provide this information and, in combination with BAL, identify correlative biomarkers useful for diagnosis and patient monitoring in blood following lung transplantation.
Conclusion
A molecular biomarker is any measurable biological molecule or signature associated with a particular physiological or pathological state that can be quantified as an indicator of predisposition, development or prognosis of diseases and response to pharmacological activity. Many recent studies targeting biomarker discovery in lung transplant rejection have emerged, but no reliable biomarker identified so far is able to diagnose or predict rejection successfully. However, the findings are promising and add knowledge to the field. Understanding the dynamics of immune cells and immune-related cytokines and chemokines provides insight into the immunological mechanisms acting during different stages of rejection and rejection phenotypes such as BOS and RAS. This information will likely shape personalized medicine and the lifestyles of patients to avoid or minimize rejection. Gene-expression studies likewise yield information furthering our understanding of mechanisms of rejection as well as serving as validation of previously published studies. Results of observational studies in BAL and blood and associated co-regulated genes will help clarify the pathways of rejection and the spectrum of phenotypes observed in rejection. CLAD is a cluster of heterogeneous phenotypes of rejection, and more research is needed in the field to untangle the mechanisms and pathobiology of these phenotypes, yet no biomarker has been successful at discriminating between phenotypes. Additionally, research needs to integrate environmental variations, clinical history and biomarkers to answer these questions.
CLAD is usually associated with long-term, gradual decline in lung function rather than rapid decline, suggesting that earlier stages of rejection need to be identified before an irreversible stage is reached. This needs to be taken into consideration when investigating biomarkers, and biomarkers should be identified that can predict time to CLAD. Detecting early decline in lung function will be crucial in developing treatments and interventions prior to irreversible decline in lung function. Early detection would mean early intervention to reduce rejection and improve the survival of lung transplant recipients.
Future perspective
All of the above-mentioned studies identify several potential biomarkers needing validation in large-scale, multicenter analyses. The aim would be to replace the current gold standard method, TBB, which is invasive, costly and prone to sampling error and inter-observer variation. Therefore, it should be emphasized that the need for reliable biomarkers to diagnose and monitor rejection is urgent and crucial to improve the current low survival rates observed in lung transplantation. A single biomarker may not be ideal; however, combining several reliable markers would be the ultimate approach to maximize the reliability and specificity for detecting rejection in the lung. Different stages of lung transplantation from organ procurement (as in organ matching) to immune monitoring of patients after lung transplantation require careful evaluation. We propose a hypothetical sequence of using potential biomarkers effectively, as illustrated in Figure 1. Similarly, studies need to focus on identifying risk factors based on a more holistic evaluation considering environmental factors, patient history, age, ethnic and geographic variations and different phenotypes and stages of rejection. This will enable clinicians to identify high- and low-risk patients and help them to treat patients based on risk with tailor-made therapies to suit the individual phenotype of the patient. Such studies would enable the curation of a precise group of biomarkers predicting graft survival in lung transplant patients. Though we are not quite there yet, ongoing studies are promising, and we are now on the brink of a breakthrough in the discovery of ideal biomarkers in lung transplant rejection.
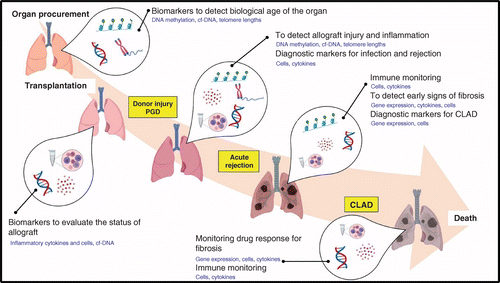
Transplanted lungs remain at risk of chronic rejection, also called chronic lung allograft dysfunction (CLAD), affecting approximately 50% of all lung transplant recipients by 5 post-operative years.
Early CLAD diagnosis or ideally prediction of CLAD is essential to enable early intervention before significant lung injury occurs.
Several biomarkers associated with CLAD have been evaluated in blood, bronchoalveolar lavage and transbronchial biopsy; however, implementation in diagnostics has not yet eventuated due to poor specificity or sensitivity and failure to detect early-stage disease.
Potential CLAD biomarkers include inflammatory proteins, immune cells, epigenetic markers, single-nucleotide polymorphisms, miRNA and cfDNA markers.
This review, while providing an update of current knowledge in the field, also emphasizes the urgent and crucial need for reliable biomarkers to diagnose and monitor rejection to improve the current low survival rates observed in lung transplantation.
A single biomarker may not be ideal, but combining several reliable markers would maximize reliability and specificity for detecting rejection in the lung.
A future challenge is integrated combinatorial biomarker machine learning to accurately predict CLAD.
Financial & competing interests disclosure
The authors wish to recognize the generosity of The Prince Charles Hospital Foundation for funding of this project via the Emerging Researcher Programme. The authors have no other relevant affiliations or financial involvement with any organization or entity with a financial interest in or financial conflict with the subject matter or materials discussed in the manuscript apart from those disclosed.
No writing assistance was utilized in the production of this manuscript.
Crown copyright
This work is licensed under Crown copyright protection and licensed for use under the Open Government Licence unless otherwise indicated. Where any of the Crown copyright information in this work is republished or copied to others, the source of the material must be identified and the copyright status under the Open Government Licence acknowledged. Published under CC-BY 4.0 www.nationalarchives.gov.uk/doc/open-government-licence/version/3/ © Crown Copyright.
Papers of special note have been highlighted as: • of interest; •• of considerable interest
References
- 1. Chronic lung allograft dysfunction: a systematic review of mechanisms. Transplantation 100(9), 1803–1814 (2016).
- 2. The International Thoracic Organ Transplant Registry of the International Society for Heart and Lung Transplantation: thirty-fifth adult lung and heart-lung transplant report-2018; focus theme: multiorgan transplantation. J. Heart Lung Transplant. 37(10), 1169–1183 (2018).
- 3. Chronic lung allograft dysfunction: definition, diagnostic criteria, and approaches to treatment – a consensus report from the Pulmonary Council of the ISHLT. J. Heart Lung Transplant. 38(5), 493–503 (2019).
- 4. . Chronic lung allograft dysfunction: evolving concepts and therapies. Semin. Respir. Crit. Care Med. 39(2), 155–171 (2018).
- 5. An international ISHLT/ATS/ERS clinical practice guideline: diagnosis and management of bronchiolitis obliterans syndrome. Eur. Respir. J. 44(6), 1479 (2014).
- 6. Chronic lung allograft dysfunction: definition and update of restrictive allograft syndrome – a consensus report from the Pulmonary Council of the ISHLT. J. Heart Lung Transplant. 38(5), 483–492 (2019).
- 7. . Early identification of chronic lung allograft dysfunction: the need of biomarkers. Front. Immunol. 10, 1681 (2019).
- 8. . Emerging biomarkers in chronic lung allograft dysfunction. Expert Rev. Mol. Diagn. 20(5), 467–475 (2020).
- 9. Bronchoalveolar lavage cytokine profile in a cohort of lung transplant recipients: a predictive role of interleukin-12 with respect to onset of bronchiolitis obliterans syndrome. J. Heart Lung Transplant. 23(9), 1053–1060 (2004).
- 10. . T regulatory cells in stable posttransplant bronchiolitis obliterans syndrome. Transplantation 84(7), 908–916 (2007).
- 11. Decreased percentage of CD4+ FoxP3+ cells in bronchoalveolar lavage from lung transplant recipients correlates with development of bronchiolitis obliterans syndrome. Transplantation 90(5), 540–546 (2010).
- 12. Association of higher CD4+ CD25highCD127low, FoxP3+, and IL-2+ T cell frequencies early after lung transplantation with less chronic lung allograft dysfunction at two years. Am. J. Transplant. 17, 1637–1648 (2017).
- 13. Analysis of long term CD4 +CD25highCD127- T-reg cells kinetics in peripheral blood of lung transplant recipients. BMC Pulm. Med. 17(1), 102 (2017).
- 14. Increased frequency of CD4(+) CD25(high) CD127(low) T cells early after lung transplant is associated with improved graft survival – a retrospective study. Transpl. Int. 33(5), 503–516 (2020).
- 15. High circulating CD4(+)CD25(hi)FOXP3(+) T-cell sub-population early after lung transplantation is associated with development of bronchiolitis obliterans syndrome. J. Heart Lung Transplant. 37(6), 770–781 (2018).
- 16. Profiling of peripheral blood mononuclear cells does not accurately predict the bronchiolitis obliterans syndrome after lung transplantation. Transpl. Immunol. 32(3), 195–200 (2015).
- 17. . Elevated bronchoalveolar lavage eosinophilia correlates with poor outcome after lung transplantation. Transplantation 97(1), 83–89 (2014).
- 18. Peripheral blood eosinophilia is associated with poor outcome post-lung transplantation. Cells 9(11), 2516 (2020).
- 19. Mathematical modeling of peripheral blood neutrophil kinetics to predict CLAD after lung transplantation. Transpl. Immunol. 62, 101321 (2020).
- 20. Early posttransplant inflammation promotes the development of alloimmunity and chronic human lung allograft rejection. Transplantation 83(2), 150–158 (2007).
- 21. Systemic and exhaled cytokine and chemokine profiles are associated with the development of bronchiolitis obliterans syndrome. J. Heart Lung Transplant. 29(9), 997–1008 (2010).
- 22. Increased levels of T cell granzyme b in bronchiolitis obliterans syndrome are not suppressed adequately by current immunosuppressive regimens. Clin. Exp. Immunol. 158(2), 230–236 (2009).
- 23. Circulating and intrapulmonary C-reactive protein: a predictor of bronchiolitis obliterans syndrome and pulmonary allograft outcome. J. Heart Lung Transplant. 28(8), 799–807 (2009).
- 24. Regulatory CD4+ CD25+ T cells in the peripheral blood of lung transplant recipients: correlation with transplant outcome. Transplantation 77(5), 762–766 (2004).
- 25. Regulatory and effector cell disequilibrium in patients with acute cellular rejection and chronic lung allograft dysfunction after lung transplantation: comparison of peripheral and alveolar distribution. Cells 10(4), 780 (2021).
- 26. Posttransplant bronchiolitis obliterates syndrome is associated with bronchialepthelial to mesenchymal transition. Am. J. Transplant. 9(4), 727–733 (2009).
- 27. International Society for Heart and Lung Transplantation consensus statement for the standardization of bronchoalveolar lavage in lung transplantation. J. Heart Lung Transplant. 39(11), 1171–1190 (2020).
- 28. Usefulness of gene expression profiling of bronchoalveolar lavage cells in acute lung allograft rejection. J. Heart Lung Transplant. 38(8), 845–855 (2019). •• Differentially expressed genes in bronchoalveolar lavage cells isolated from chronic lung allograft dysfunction and chronic lung allograft dysfunction-free patients.
- 29. . Bronchoalveolar lavage. In: Principles and Practice of Interventional Pulmonology. Ernst AHerth FJF (Eds). Springer Science + Business Media, NY, USA (2013).
- 30. . Description of an automated method for urea nitrogen determination in bronchoalveolar lavage fluid (BALF) of neonates and infants. J. Lab. Autom. 20(6), 636–641 (2015).
- 31. Heparin-binding protein, lysozyme, and inflammatory cytokines in bronchoalveolar lavage fluid as diagnostic tools for pulmonary infection in lung transplanted patients. Am. J. Transplant. 18(2), 444–452 (2018).
- 32. Protection against bronchiolitis obliterans syndrome is associated with allograft CCR7+ CD45RA- T regulatory cells. PLoS ONE 5(6), e11354 (2010).
- 33. Humoral immunity in phenotypes of chronic lung allograft dysfunction: a broncho-alveolar lavage fluid analysis. Transpl. Immunol. 38, 27–32 (2016).
- 34. The role of the IL23/IL17 axis in bronchiolitis obliterans syndrome after lung transplantation. Am. J. Transplant. 8(9), 1911–1920 (2008).
- 35. Association of methacholine challenge test with diagnosis of chronic lung allograft dysfunction in lung transplant patients. Clin. Transplant. 32(10), e13397 (2018).
- 36. Prognostic value of bronchoalveolar lavage neutrophilia in stable lung transplant recipients. J. Heart Lung Transplant. 28(5), 468–474 (2009).
- 37. BALF cytokines in different phenotypes of chronic lung allograft dysfunction in lung transplant patients. Clin. Transplant. 31(3), (2017).
- 38. Differential cytokine, chemokine and growth factor expression in phenotypes of chronic lung allograft dysfunction. Transplantation 99(1), 86–93 (2015).
- 39. Mechanistic differences between phenotypes of chronic lung allograft dysfunction after lung transplantation. Transpl. Int. 27(8), 857–867 (2014).
- 40. Role of cytokine profile in the differential diagnosis between acute lung rejection and pulmonary infections after lung transplantation. Eur. J. Cardiothorac. Surg. 47(6), 1031–1036 (2015).
- 41. . Bronchoalveolar lavage fluid characteristics in acute and chronic lung transplant rejection. J. Heart Lung Transplant. 23(5), 532–540 (2004).
- 42. . Elevated levels of interleukin-8 and transforming growth factor-beta in bronchoalveolar lavage fluid from patients with bronchiolitis obliterans syndrome: proinflammatory role of bronchial epithelial cells. Transplantation 70, 362–367 (2000).
- 43. Upregulation of chemokines in bronchoalveolar lavage fluid as a predictive marker of post-transplant airway obliteration. J. Heart Lung Transplant. 21(7), 721–730 (2002).
- 44. The role of TGF-beta in the association between primary graft dysfunction and bronchiolitis obliterans syndrome. Am. J. Transplant. 16(2), 640–649 (2016).
- 45. . Oxidative stress and DNA methylation regulation in the metabolic syndrome. Epigenomics 7(2), 283–300 (2015).
- 46. . The epigenetic clock as a predictor of disease and mortality risk: a systematic review and meta-analysis. Clin. Epigenetics 11(1), 62 (2019).
- 47. . DNA methylation age of human tissues and cell types. Genome Biology 14(10), R115 (2013).
- 48. Altered DNA methylation profile in idiopathic pulmonary fibrosis. Am. J. Respir. Crit. Care Med. 186(6), 525–535 (2012).
- 49. Global methylation patterns in idiopathic pulmonary fibrosis. PloS One 7(4), e33770 (2012).
- 50. . More than a genetic code: epigenetics of lung fibrosis. Mol. Diagn. Ther. 24(6), 665–681 (2020).
- 51. Newborn DNA-methylation, childhood lung function, and the risks of asthma and COPD across the life course. Eur. Respir. J. 53(4), 1801795 (2019).
- 52. Identification of novel differentially methylated sites with potential as clinical predictors of impaired respiratory function and COPD. EBioMedicine 43, 576–586 (2019).
- 53. . The epigenetic promise to improve prognosis of heart failure and heart transplantation. Transplant. Rev. 31, 249–256 (2017).
- 54. Unveiling the role of DNA methylation in kidney transplantation: novel perspectives toward biomarker identification. BioMed Res. Int. 2019, 1602539 (2019).
- 55. . The role of bronchoscopic surveillance monitoring in the care of lung transplant recipients. Semin. Respir. Crit. Care Med. 27(5), 480–491 (2006).
- 56. Reliability for grading acute rejection and airway inflammation after lung transplantation. J. Heart Lung Transplant. 24(6), 652–657 (2005).
- 57. . Bronchoalveolar lavage cell gene expression in acute lung rejection: development of a diagnostic classifier. Transplantation 85(2), 224–231 (2008).
- 58. The role of interleukin-17 during acute rejection after lung transplantation. Eur. Respir. J. 27(4), 779–787 (2006).
- 59. . Aspiration, localized pulmonary inflammation, and predictors of early-onset bronchiolitis obliterans syndrome after lung transplantation. J. Am. Coll. Surg. 217(1), 90–100; discussion 100–101 (2013).
- 60. CXCR3 and its ligand CXCL10 are expressed by inflammatory cells infiltrating lung allografts and mediate chemotaxis of T cells at sites of rejection. Am. J. Pathol. 158(5), 1703–1711 (2001).
- 61. The prognostic importance of bronchoalveolar lavage fluid CXCL9 during minimal acute rejection on the risk of chronic lung allograft dysfunction. Am. J. Transplant. 18(1), 136–144 (2018).
- 62. . Acute lung transplant rejection is associated with localized increase in T-cell IFNgamma and TNFalpha proinflammatory cytokines in the airways. Transplantation 84(11), 1452–1458 (2007).
- 63. Blood gene expression predicts bronchiolitis obliterans syndrome. Front. Immunol. 8, 1841 (2018). •• Differentially expressed genes in blood cells in bronchiolitis obliterans syndrome and stable patients, with expression levels enabling stratification of patients based on lung rejection risk.
- 64. A common rejection module (CRM) for acute rejection across multiple organs identifies novel therapeutics for organ transplantation. J. Exp. Med. 210(11), 2205–2221 (2013).
- 65. The common rejection module in chronic rejection post lung transplantation. PLoS ONE 13(10), e0205107 (2018).
- 66. Banff 2019 meeting report: molecular diagnostics in solid organ transplantation – consensus for the Banff Human Organ Transplant (B-HOT) gene panel and open source multicenter validation. Am. J. Transplant. 20(9), 2305–2317 (2020). • NanoString technology, including automated platform, for routine diagnostic workflow to detect 770 validated genes associated with organ rejection, tissue damage and immune responses.
- 67. HLA-G*01:04∼UTR3 recipient correlates with lower survival and higher frequency of chronic rejection after lung transplantation. Am. J. Transplant. 15(9), 2413–2420 (2015).
- 68. Immunohistochemical study of HLA-G expression in lung transplant recipients. Am. J. Transplant. 9(6), 1427–1438 (2009).
- 69. . Association of soluble HLA-G with acute rejection episodes and early development of bronchiolitis obliterans in lung transplantation. PLoS One 9(7), e103643 (2014).
- 70. HLA-E(*)01:03 allele in lung transplant recipients correlates with higher chronic lung allograft dysfunction occurrence. J. Immunol. Res. 2016, 1910852 (2016).
- 71. Impacts of single-nucleotide polymorphisms in Fc gamma receptor IIA (rs1801274) on lung transplant outcomes among Japanese lung transplant recipients. Transpl. Int. 34(11), 2192–2204 (2021).
- 72. FCGR3A and FCGR2A genotypes differentially impact allograft rejection and patients' survival after lung transplant. Front. Immunol. 10, 1208 2019).
- 73. Interleukin-17 receptor polymorphism predisposes to primary graft dysfunction after lung transplantation. J. Heart Lung Transplant. 34(7), 941–949 (2015).
- 74. Dectin-1 genetic deficiency predicts chronic lung allograft dysfunction and death. JCI Insight 4(22), e133083 (2019).
- 75. Dysregulated microRNA expression and chronic lung allograft rejection in recipients with antibodies to donor HLA. Am. J. Transplant. 15(7), 1933–1947 (2015).
- 76. MicroRNA 10a marks regulatory T cells. PLoS ONE 7(5), e36684 (2012).
- 77. Expression of miRNAs miR-133b and miR-206 in the Il17a/f locus is co-regulated with IL-17 production in αβ and χδ T cells. PLoS ONE 6(5), e20171 (2011).
- 78. Cell-free DNA and active rejection in kidney allografts. J. Am. Soc. Nephrol. 28(7), 2221–2232 (2017).
- 79. Liquid biopsies come of age: towards implementation of circulating tumour DNA. Nat. Rev. Cancer 17, 223–238 (2017).
- 80. . Donor-derived cell-free DNA in solid-organ transplant diagnostics: indications, limitations, and future directions. Transplantation 105(6), 1203–1211 (2021).
- 81. Donor-derived cell-free DNA predicts allograft failure and mortality after lung transplantation. EBioMedicine 40, 541–553 (2019). • A proof-of-concept study to use cell-free DNA to predict lung transplant outcome as a less invasive alternative to lung biopsies.
- 82. Cell-free DNA and CXCL10 derived from bronchoalveolar lavage predict lung transplant survival. J. Clin. Med. 8(2), 241 (2019). • Combination of cell-free DNA and CXCL10 levels in bronchoalveolar lavage to stratify patients into chronic lung allograft dysfunction phenotypes (bronchiolitis obliterans syndrome and restrictive allograft syndrome) and stable.
- 83. . Characteristics, properties, and potential applications of circulating cell-free DNA in clinical diagnostics: a focus on transplantation. J. Immunol. Methods 463, 27–38 (2018).
- 84. Comprehensive human cell-type methylation atlas reveals origins of circulating cell-free DNA in health and disease. Nat. Commun. 9(1), 5068 (2018).
- 85. . HLA mismatches influence lung transplant recipient survival, bronchiolitis obliterans and rejection: implications for donor lung allocation. J. Heart Lung Transplant. 30(4), 426–434 (2011).
- 86. Influence of human leukocyte antigen mismatching on bronchiolitis obliterans syndrome in lung transplantation. J. Heart Lung Transplant. 35(2), 186–194 (2016).
- 87. Impact of human leukocyte antigen mismatch on lung transplant outcome. Interact. Cardiovasc. Thorac. Surg. 26(5), 859–864 (2018).
- 88. Relative impact of human leukocyte antigen mismatching and graft ischemic time after lung transplantation. J. Heart Lung Transplant. 27(6), 628–634 (2008).
- 89. Long-term outcome of lung transplantation is predicted by the number of HLA-DR mismatches. Transplantation 71(3), 368–373 (2001).
- 90. The Munich-LTX-Score: predictor for survival after lung transplantation. Clin. Transplant. 26(1), 173–183 (2012).
- 91. Pre-transplant panel reactive antibody in lung transplant recipients is associated with significantly worse post-transplant survival in a multicenter study. J. Heart Lung Transplant. 24(Suppl. 7), S249–S254 (2005).
- 92. . Pretransplant panel reactive antibodies in human lung transplantation: an analysis of over 10,000 patients. Ann. Thorac. Surg. 85(6), 1919–1924 (2008).
- 93. The impact of pre-transplant allosensitization on outcomes after lung transplantation. J. Heart Lung Transplant. 34, 1415–1422 (2015).
- 94. A virtual crossmatch-based strategy for perioperative desensitisation in lung transplant recipients with preformed donor-specific antibodies: 3-year outcome. Eur. Respir. J.
doi:10.1183/13993003.04090-2020 (2021) (Epub ahead of print). - 95. Donor-specific and -nonspecific HLA antibodies and outcome post lung transplantation. Eur. Respir. J. 50(5), 1701248 (2017).
- 96. Development of an antibody specific to major histocompatibility antigens detectable by flow cytometry after lung transplant is associated with bronchiolitis obliterans syndrome. Transplantation 74(6), 799–804 (2002).
- 97. Development of antibodies to human leukocyte antigen precedes development of antibodies to major histocompatibility class I-related chain A and are significantly associated with development of chronic rejection after human lung transplantation. Hum. Immunol. 71(6), 560–565 (2010).
- 98. De novo donor-specific HLA antibodies are associated with early and high-grade bronchiolitis obliterans syndrome and death after lung transplantation. J. Heart Lung Transplant. 33(12), 1288–1294 (2014).
- 99. Early donor-specific antibodies in lung transplantation: risk factors and impact on survival. J. Heart Lung Transplant. 33(12), 1255–1263 (2014).
- 100. De-novo donor-specific anti-HLA antibodies 30 days after lung transplantation are associated with a worse outcome. J. Heart Lung Transplant. 35(9), 1067–1077 (2016).
- 101. De novo DQ donor-specific antibodies are associated with chronic lung allograft dysfunction after lung transplantation. Am. J. Respir. Crit. Care Med. 194(5), 596–606 (2016).
- 102. Impact of pretransplant anti-HLA antibodies on outcomes in lung transplant candidates. Am. J. Respir. Crit. Care Med. 189(10), 1234–1239 (2014).
- 103. Donor-specific antibody characteristics, including persistence and complement-binding capacity, increase risk for chronic lung allograft dysfunction. J. Heart Lung Transplant. 39(12), 1417–1425 (2020).
- 104. Role of C1q-binding anti-HLA antibodies as a predictor of lung allograft outcome. Eur. Respir. J. 52(2), 1701898 (2018).
- 105. The allograft injury marker CXCL9 determines prognosis of anti-HLA antibodies after lung transplantation. Am. J. Transplant. 22(2), 16827 (2021).
- 106. Pre-transplant antibodies to Kα1 tubulin and collagen-V in lung transplantation: clinical correlations. J. Heart Lung Transplant. 32(8), 807–814 (2013).
- 107. . Antibodies to K-α 1 tubulin and collagen V are associated with chronic rejection after lung transplantation. Am. J. Transplant. 12(8), 2164–2171 (2012).
- 108. Antibodies to self-antigens predispose to primary lung allograft dysfunction and chronic rejection. Ann. Thorac. Surg. 90(4), 1094–1101 (2010).
- 109. . Prevalence of antibodies to lung self-antigens (Kα1 tubulin and collagen V) and donor specific antibodies to HLA in lung transplant recipients and implications for lung transplant outcomes: single center experience. Transpl. Immunol. 54, 65–72 (2019).
- 110. Alloimmunity-induced autoimmunity as a potential mechanism in the pathogenesis of chronic rejection of human lung allografts. J. Heart Lung Transplant. 30(6), 624–631 (2011).
- 111. . Exosomes: from cell debris to potential biomarkers in transplantation. Transplantation 101(10), 2275–2276 (2017).
- 112. . Emerging role of exosomes in allorecognition and allograft rejection. Curr. Opin. Organ Transplant. 23(1), 22–27 (2018).
- 113. . Circulating exosomes with distinct properties during chronic lung allograft rejection. J. Immunol. 200(8), 2535–2541 (2018).
- 114. Donor-derived exosomes with lung self-antigens in human lung allograft rejection. Am. J. Transplant. 17(2), 474–484 (2017).
- 115. . The role of exosomes in allograft immunity. Cell. Immunol. 331, 85–92 (2018).
- 116. The role of donor-derived exosomes in lung allograft rejection. Hum. Immunol. 80(8), 588–594 (2019).
- 117. Decline in club cell secretory proteins, exosomes induction and immune responses to lung self-antigens, Kalpha1 tubulin and collagen V, leading to chronic rejection after human lung transplantation. Transplantation 105(6), 1337–1346 (2021).
- 118. Global proteomics analysis of circulating extracellular vesicles isolated from lung transplant recipients. ACS Omega 5(24), 14360–14369 (2020).
- 119. Lung microbiota predict chronic rejection in healthy lung transplant recipients: a prospective cohort study. Lancet Respir. Med. 9(6), 601–612 (2021).
- 120. Importance of the preoperative prognostic nutritional index score as a predictor of chronic lung allograft dysfunction after lung transplantation: a Japanese single-institution study. Surg. Today 51(12), 1946–1952 (2021).
- 121. Multi-omics profiling predicts allograft function after lung transplantation. Eur. Respir. J 59(2):2003292 2003292 (2021).
- 122. . Understanding the odd science of aging. Cell 120(4), 437–447 (2005).
- 123. . The hallmarks of aging. Cell 153(6), 1194–1217 (2013).
- 124. . Rate of normal lung function decline in ageing adults: a systematic review of prospective cohort studies. BMJ Open 9(6), e028150 (2019).
- 125. . Telomere shortening in human diseases. FEBS J. 280(14), 3180–3193 (2013).
- 126. . Therapeutic targeting of telomerase. Genes 7(7), 39 (2016).
- 127. Exome sequencing links mutations in PARN and RTEL1 with familial pulmonary fibrosis and telomere shortening. Nat. Genet. 47(5), 512–517 (2015).
- 128. Association between donor leukocyte telomere length and survival after unrelated allogeneic hematopoietic cell transplantation for severe aplastic anemia. JAMA 313(6), 594–602 (2015).
- 129. Effect of delayed graft function, acute rejection and chronic allograft dysfunction on kidney allograft telomere length in patients after transplantation: a prospective cohort study. BMC Nephrol. 16, 23 (2015).
- 130. Association of donor and recipient telomere length with clinical outcomes following lung transplantation. PLoS ONE 11(9), e0162409 (2016).
- 131. . Telomere length in patients with pulmonary fibrosis associated with chronic lung allograft dysfunction and post-lung transplantation survival. J. Heart Lung Transplant. 36(8), 845–853 (2017).
- 132. Shorter telomere length following lung transplantation is associated with clinically significant leukopenia and decreased chronic lung allograft dysfunction-free survival. ERJ Open Res. 6(2), 00003–02020 (2020).
- 133. Short lung transplant donor telomere length is associated with decreased CLAD-free survival. Thorax 72(11), 1052–1054 (2017). •• Short telomeres are a biomarker of chronic lung allograft dysfunction in a substantial cohort of lung transplant recipients.
- 134. . Airway telomere length in lung transplant recipients. Front. Immunol. 12, 658062 (2021).
- 135. . Where the chromosome ends: telomeres and cytomegalovirus risk in lung transplant recipients. Am. J. Respir. Crit. Care Med. 199(3), 265–267 (2019).
- 136. Lung transplant outcomes in patients with pulmonary fibrosis with telomere-related gene variants. Chest 156(3), 477–485 (2019).
- 137. Resident cellular components of the human lung: current knowledge and goals for research on cell phenotyping and function. Proc. Am. Thorac. Soc. 5, 763–766 (2008).
- 138. A molecular cell atlas of the human lung from single-cell RNA sequencing. Nature 587(7835), 619–625 (2020).
- 139. single-cell RNA-seq reveals ectopic and aberrant lung resident cell populations in idiopathic pulmonary fibrosis. Sci. Adv. 6(28), eaba1983 (2020).
- 140. Single-cell RNA-sequencing reveals profibrotic roles of distinct epithelial and mesenchymal lineages in pulmonary fibrosis. Sci. Adv. 6(28), eaba1972 (2020).
- 141. Single-cell transcriptomic analysis of human lung reveals complex multicellular changes during pulmonary fibrosis.
doi:10.1101/296608 (2018) (Epub ahead of print). - 142. Single-cell RNA sequencing of the T helper cell response to house dust mites defines a distinct gene expression signature in airway Th2 cells. Immunity 51, 169–184 (2019).
- 143. Single-cell transcriptomic analysis of human lung provides insights into the pathobiology of pulmonary fibrosis. Am. J. Respir. Crit. Care Med. 199(12), 1517–1536 (2019).
- 144. Proliferating SPP1/MERTK-expressing macrophages in idiopathic pulmonary fibrosis. Eur. Respir. J. 54(2), 1802441 (2019).
- 145. Dynamics of human monocytes and airway macrophages during healthy aging and after transplant. J. Exp. Med. 217(3), e20191236 (2020). • Single-cell RNA sequencing technology unraveling the replacement of donor immune cells in transplanted lungs, demonstrating the feasibility of single-cell RNA sequencing to understand the pathogenesis of chronic lung allograft dysfunction, and a future resource for biomarker discovery.
- 146. The autotaxin-lysophosphatidic-acid pathway mediates mesenchymal cell recruitment and fibrotic contraction in lung transplant fibrosis. J. Heart Lung Transplant. 40(1), 12–23 (2021).